Chapter 13 PET studies demonstrated a global decrease of CMRglu (>40%) during non-REM sleep as compared to wakefulness. At the regional level, mostly decreases of CMRglu and rCBF were observed during non-REM sleep, in various subcortical structures and associative cortical areas. REM sleep, on the other hand, displayed a global CMRglu as high as during wakefulness. Locally, increases and decreases of CMRglu and rCBF were reported in areas responsible for the generation of REM sleep and some important characteristics of dreams. Beyond sleep stages, fMRI studies described neural responses associated with brain oscillations of sleep: spindles and slow waves during non-REM sleep, and ponto-geniculo-occipital waves during REM sleep. Besides refining the characterization of sleep functional neuroanatomy, fMRI data also demonstrated that non-REM sleep is not merely a passive state of brain deactivation, by showing enhanced brain responses associated with spindles and slow waves. At the subcortical level, localized decreases of rCBF during non-REM sleep, as compared to wakefulness and REM sleep, were observed in the brainstem, thalamus, basal ganglia, and basal forebrain.1 These structures include groups of neurons critical for the modulation of arousal and the generation of spontaneous neural oscillations of sleep. At the cortical level, although rCBF in primary cortices remained relatively preserved, decreases were located in several associative areas: (medial) prefrontal cortex, anterior and posterior cingulate gyrus, and precuneus. These areas are highly active in wakefulness, during which they are involved in complex cognitive processes and also participate in the modulation of non-REM sleep oscillations (see later). A representation of these PET results on non-REM sleep is provided in Figure 13.1. FIGURE 13.1 Functional neuroanatomy of normal human non–rapid eye movement (non-REM) sleep (H215O positron emission tomography). Brain areas in which regional cerebral blood flow decreases during non-REM sleep as compared to wakefulness and REM sleep. Image sections are displayed on different levels of the z-axis as indicated at the top of each image. The color scale indicates the range of Z values for the activated voxels, superimposed on a canonical T1-weighted magnetic resonance image. Displayed voxels are significant at P < .05 after correction for multiple comparisons. (Modified from Maquet P, Degueldre C, Delfiore G, et al. Functional neuroanatomy of human slow wave sleep. J Neurosci. 1997;17:2807-2812 with permission.) Within non-REM sleep, fMRI studies reported brain activations associated with the major neural rhythms that define this sleep stage: spindles and slow waves. Spindles—prominent during stage N2—were correlated with increased brain responses in the lateral and posterior aspects of the thalamus, in agreement with the central role of several thalamic nuclei (reticular thalamic and thalamocortical neurons) in the generation of spindles.2 Spindles were also associated with activation of specific paralimbic (anterior cingulate cortex, insula) and neocortical (superior temporal gyrus) areas. The hypothesis of two spindle subtypes—slow (11 to 13 Hz) and fast (13 to 15 Hz)—with distinct neural correlates was also explored with fMRI. It was observed that slow spindles exhibited a network very close to the “total” spindle one. In contrast, fast spindles were associated with a more diffuse cortical activation extending to somatosensory areas and mid–cingulate cortex. These neural correlates of sleep spindles with fMRI are shown in Figure 13.2. Slow waves, on the other hand, were associated with enhanced brain responses in inferior and medial aspects of the prefrontal cortex, a major site for slow wave initiation as corroborated by electrophysiological data. Other activations correlated with slow waves were located in parahippocampal gyrus, precuneus, posterior cingulate cortex, cerebellum, and brainstem.3 The neural correlates of sleep slow waves are summarized in Figure 13.3. Altogether these fMRI studies have identified brain regions involved in the generation, propagation, or modulation of non-REM sleep oscillations. FIGURE 13.2 Functional neuroanatomy of sleep spindles (functional magnetic resonance imaging). FIGURE 13.3 Functional neuroanatomy of sleep slow waves (functional magnetic resonance imaging). Combined with neuropsychological findings in brain-damaged patients, those patterns might also explain several hallmarks of dreaming experience that are found in dream reports after awakening from REM sleep.4 For example, the increased activity in the amygdala is consistent with the predominance of threat-related emotions in dreams. Temporo-occipital increased activity is in keeping with visual dream imagery, which constitutes most of the sensorial experiment while dreaming. Also, prefrontal deactivation is evocative of the alteration in time perception, the lack of orientational stability, the delusional belief of being awake, the fragmented episodic memory recall, and the decrease in volitional control experienced in dreams. Finally, inferior parietal deactivation might contribute to the lack of distinction between first- and third-person perspectives, which is a core characteristic of the dream scenario. A summary of the neural correlates of REM sleep and dreaming is provided in Figure 13.4. FIGURE 13.4 Functional neuroanatomy of rapid eye movement (REM) sleep and dreaming (positron emission tomography). Schematic representation of the relative decreases (in blue) and increases (in red) in regional cerebral blood flow or cerebral metabolic rate of glucose associated with REM sleep. Arrows show the proposed relationships between several dreaming features and cerebral regions associated with REM sleep. The upper panel displays a lateral view; the right lower panel displays a medial view; the left lower panel displays a ventral view. A, Amygdala; B, basal forebrain; Ca, anterior cingulate gyrus; Cp, posterior cingulate gyrus and precuneus; F, prefrontal cortex (middle, inferior, and orbitofrontal cortices); H, hypothalamus; M, motor cortex; O, occipital-lateral cortex; P, parietal cortex (inferior parietal lobule); PH, parahippocampical gyrus; Th, thalamus; T-O, temporo-occipital extrastriate cortex; TP, pontine tegmentum. (Modified from Schwartz S, Maquet P. Sleep imaging and the neuro-psychological assessment of dreams. Trends Cogn Sci. 2002;6:23-30. Copyright 2002, with permission from Elsevier.) Neuroimaging studies have particularly addressed the neuropsychological damages induced by OSA. Structural alterations were found in the prefrontal cortex, hippocampal and parietal cortex in OSA patients before treatment, compared to controls. In these regions MRI using VBM found decreases of gray matter.5 Several of those abnormal patterns were, however, reversible under continuous positive airway pressure (CPAP) treatment for 3 months. Structural changes were paralleled by impairments in various cognitive functions, which were improved after CPAP. Interestingly, posttreatment improvements in neuropsychological scores were correlated with posttreatment increases in gray matter, particularly in the hippocampus (Fig. 13.5). FIGURE 13.5 Structural brain changes in obstructive sleep apnea (OSA) before and after continuous positive airway pressure treatment (voxel-based morphometry). Although the fundamental pathophysiological mechanisms are not fully understood, a central dysregulation of the autonomic nervous system might participate in these processes. For instance, during respiratory maneuvers (Valsalva), altered fMRI signals were shown in OSA patients compared to controls. These abnormal activations were located in the cerebellar cortex and deep nuclei; superior frontal, precentral, cingulate, inferior parietal, superior temporal, and insular cortices; hippocampus; and midbrain. These data suggest a dysfunction of neural structures integrating afferent airway signals with autonomic and somatic responses.6 It is important to notice that peripheral factors might confound the deficits observed in studies focused on OSA patients, including, for example, exaggerated body mass index and motivational problems. Interestingly, overlaps of structural and functional deficits in the hippocampus, anterior cingulate, and frontal cortex (areas consistently showing abnormal structure or function in the depression literature) provide several potential biological links between OSA and mood disorders.
Neuroimaging Techniques
Normal Sleep
Non-REM Sleep
Brain responses associated with all sleep spindles (i.e., slow and fast; center panels, F to I), slow spindles (left panels, A to E), and fast spindles (right panels, J to M), as compared to the baseline brain activity of non-REM sleep. Activations were significant at P < .05, corrected for multiple comparisons. The side panels show the time course (in seconds) of fitted response amplitudes (in arbitrary units) during spindles in the corresponding circled brain area. All responses consisted of regional increases of brain activity. (Modified from Schabus M, Dang-Vu TT, Albouy G, et al. Hemodynamic cerebral correlates of sleep spindles during human non-rapid eye movement sleep. Proc Natl Acad Sci U S A. 2007;104:13164-13169. Copyright 2007 National Academy of Sciences, U.S.A.)
Brain responses associated with slow waves, as compared to the baseline brain activity of non-REM sleep. Brain responses were significant at P < .05, corrected for multiple comparisons. Activations were located in the pons (A), cerebellum (B), parahippocampal gyrus (C), inferior frontal gyrus (D), precuneus (E), and posterior cingulate gyrus (F). The side panels show the time course (in seconds) of fitted response amplitudes (in arbitrary units) during slow waves in the corresponding circled brain area. All responses consisted of regional increases of brain activity. (Modified from Dang-Vu TT, Schabus M, Desseilles M, et al. Spontaneous neural activity during human slow wave sleep. Proc Natl Acad Sci U S A. 2008;105:15160-15165. Copyright 2008 National Academy of Sciences, U.S.A.)
REM Sleep and Dreaming
Obstructive Sleep Apnea
Top row, Regions showing a gray matter volume decrease in untreated patients with OSA compared with control subjects (P < .05 corrected for multiple comparisons based on cluster extent). Middle row, Regions showing a gray matter increase after treatment, compared to before treatment, in patients with OSA (P < .05 corrected for multiple comparisons based on cluster extent). Bottom row, Left hippocampal entorhinal cortex showing both a gray matter reduction before treatment and a gray matter increase after treatment (P < .001 uncorrected, based on a priori hypotheses) and the correlation between gray matter volume increase in this region and cognitive improvement after treatment (P < .05 corrected for multiple comparisons with false discovery rate). (From Canessa N, Castronovo V, Cappa SF, et al. Obstructive sleep apnea: brain structural changes and neurocognitive function before and after treatment. Am J Respir Crit Care Med. 2011;183:1419-1426. Copyright 2011, with permission from the American Thoracic Society.)
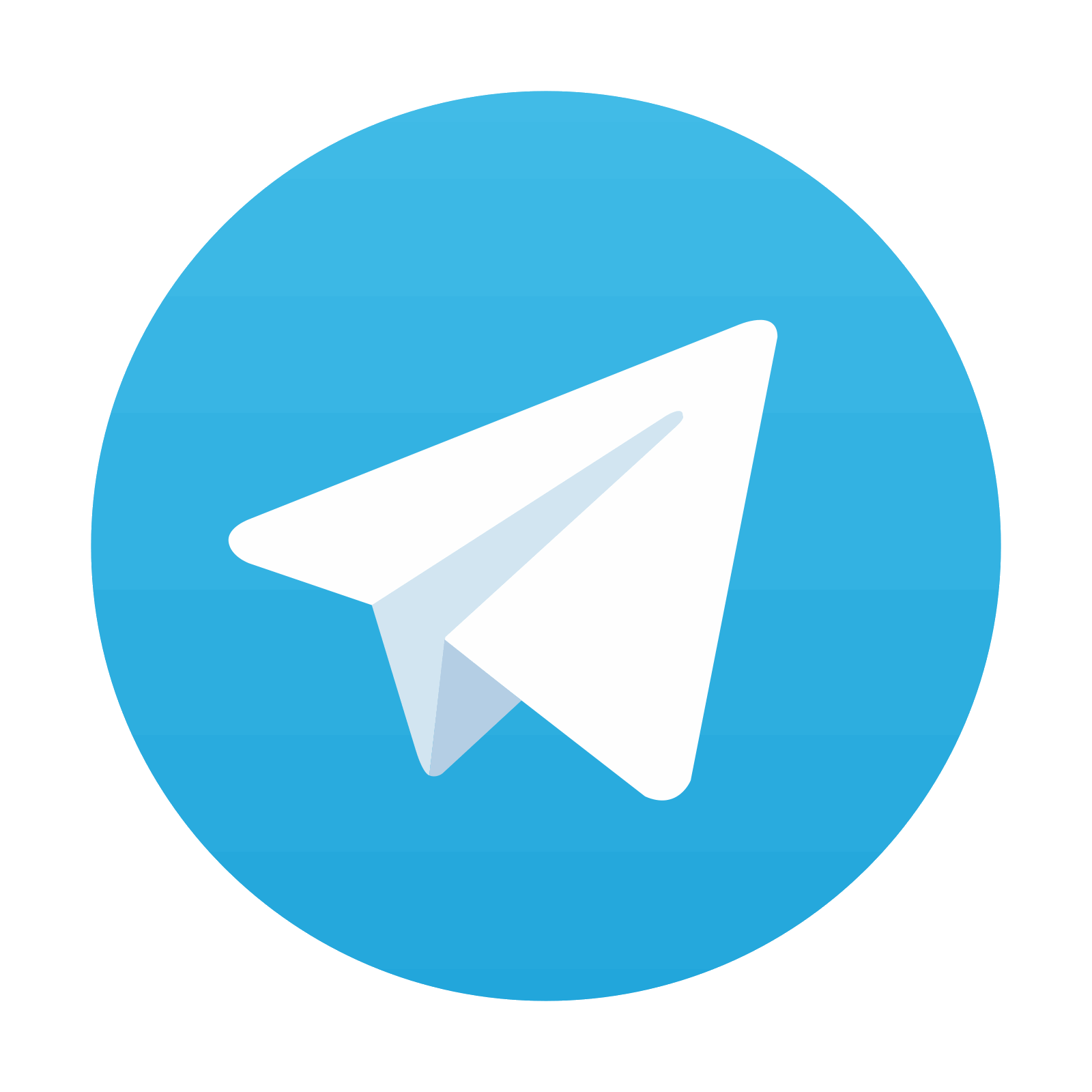