1 Anatomy of the Developing Pediatric Skull Base
Abstract
Development of the pediatric skull base is an intricate process that starts in utero and spans the entire childhood. During development, endonasal endoscopic corridors change constantly. Some skull base targets are only accessible during the late stages of development, while others are safer to access in the early stages. Importantly, care must be taken not to transgress growth centers, as this will irreversibly disrupt the developmental process. Skull base growth is an asynchronous process, with delayed growth of its anterior portions. Thus, certain anterior corridors become available only once growth stages of the respective portion of the skull base are completed. The trajectories of important neurovascular structures undergo continuous alterations under the driving force of sphenoid sinus pneumatization, further impacting the surgical corridors for endoscopic endonasal approaches. Furthermore, pathology of the skull base, depending on its location and type, will have different effects on endonasal endoscopic corridors as the developmental process is delayed or distorted. Reconstructing the skull base with a nasoseptal flap after extended endoscopic endonasal approaches is only possible after the age of 10 years, or even later in children with long-standing pathology and delayed development. Thus, understanding the different steps of skull base development, as well as the impact of skull base pathology on this process should be an integral part in the surgical planning of endoscopic endonasal approaches for pediatric patients harboring skull base lesions.
1.1 Introduction
Development of the skull base begins at approximately 4 weeks in utero and continues throughout childhood. During development, the pediatric skull base is constantly molded from above, through growth of the cranial vault and from below, through aeration of the paranasal sinuses. This will directly influence growth of the craniofacial region’ as well as trajectories of critical neurovascular structures. It is an asynchronous process, with the posterior skull base, a mesodermal structure, undergoing an accelerated growth process in early childhood, while the anterior portion, originating from neural crest cells, along with the midfacies, undergoes a growth spurt during puberty and finalizes the process after 14 years. Pneumatization of the sphenoid sinus begins at 2 years and is the main driving force of skull base development. Pneumatization of the sphenoid sinus also impacts the most important restriction site for endoscopic endonasal approaches (EEA), the intercarotid distance (ICD) at the level of the cavernous sinus, which reaches adult levels after 9 years. As the skull base grows in craniocaudal and anteroposterior direction, distances to specific target sites, such as the sella or the dens, have an abrupt growth trend, also directly correlated with the pneumatization process. The nasal septum reaches an adequate developmental stage for EEA around 10 years. At this age, the nasoseptal flap will have the appropriate length to cover defects in the anterior skull base and sellar region. The nasoseptal flap may be insufficient for repair of transclival defects in the pediatric population, regardless of age group. Finally, certain tumors, such as suprasellar craniopharyngiomas can disrupt growth centers and significantly delay the developmental process. These aspects, along with certain technical aspects related to endonasal endoscopic surgery on the developing skull base, will be detailed in this chapter.
1.2 Considerations on Surgical Approaches to the Developing Skull Base
Pathology of the pediatric skull base is largely comprised of developmental anomalies. Skull base tumors are relatively uncommon in the pediatric population but can be particularly problematic due to the disruptive effects on the normal developmental process with inherent malformations and impact on long-term prognosis. 1 , 2 Invasive surgical procedures, open microsurgical approaches, and endoscopic procedures are generally reserved for severe debilitating pathology given the additional risk of transgressing growth centers and delaying the developmental process. Irreversible effects on skull base development can occur both due to tumor growth and as a result of aggressive surgical intervention. Finally, anatomical landmarks and restriction sites for surgical approaches change constantly during childhood as a result of the developing skull base. This will have a direct impact on surgical corridors, as well as surgical tools and instruments, which should be adjusted for age group as well as developmental stage. Therefore, knowing and understanding the developmental processes and patterns of the pediatric cranial base is essential in deciding the appropriate therapeutic approach as well as in planning surgical trajectories for pediatric skull base lesions.
Many lesions in the pediatric cranial base can lead to severe functional impairment. 2 , 3 Early intervention may help reset the growth process, thereby avoiding permanent damage. 4 However, accessing the skull base is not trivial in children. The cranial fossae are significantly smaller and shallower, while critical neurovascular structures are in close proximity to each other, making access particularly challenging. 2 , 4 , 5 , 6 The stage of tooth eruption or the aeration level of the paranasal sinuses can further hinder approaches, both transfacial approaches and endonasal interventions, and should be taken into consideration when deciding the type of surgical approach. 2 , 6
In recent years, EEA have been advocated as the procedures of choice in this patient population. Feasibility of these approaches in children has been demonstrated in several modern case series. 7 , 8 , 9 , 10 , 11 , 12 Given its intrinsic magnification, the endoscope can provide superior visualization, an essential feature given the minute size of anatomical structures in children. Furthermore, endoscopic approaches can adapt more easily to the narrow anatomical corridors, thereby safely reaching areas that have thus far not been amenable to classical open routes. 5 Surgical interventions on the developing skull base can lead to disfigurement. Endonasal approaches are believed to have improved cosmetic outcomes, although inadvertent disruption of growth centers through endonasal routes can also lead to long-term disability. Navigation of growth centers during endoscopic surgery remains a major challenge. Knowledge of developmental patterns and age trends can be essential in perioperative planning for EEA.
The small pediatric skull base offers insufficient working space to accommodate both endoscope and instruments. Transitioning endoscopic approaches to the pediatric population has been delayed due to the inadequate working corridors. Choosing the appropriate trajectory to maximize your working space is a key factor in pediatric EEA and is largely based on developmental patterns that have emerged from recent radioanatomical studies. Several measurement systems have been developed over the last decade. 4 , 10 , 13 , 14 Quantitative parameters can guide preoperative planning, establishing optimal surgical routes, and anatomical limitations for the transsellar as well as expanded endoscopic approaches. Limitations for EEA corridors are primarily based on neurovascular structures with a constantly changing position during childhood. 5 Furthermore, certain anatomical structures can be reached more easily at certain points in skull base development, while other targets can only be safely accessed in the adult population.
Adequate closure of skull base defects after EEA is of utmost importance. The nasoseptal flap has been considered the most efficient method of reconstruction with a significant decline in cerebrospinal fluid (CSF) leak rates, both in the adult and in the pediatric patient population. The nasoseptal flap, a pedicled flap derived from the nasal septum and based on the nasoseptal artery, has been part of most multilayer reconstruction techniques. Development of the pediatric skull base is not a linear process. Certain parts of the skull base and the maxillofacial skeleton have a slower evolution. Importantly, skull base development appears to be accelerated in the first years of life, whereas parts of the nasal cavity lag behind. Thus, in certain age groups, especially in early childhood, the length or width of the harvested nasoseptal flap might be insufficient to repair large skull base defects. It is therefore important to have a complete understanding of the developmental process of both the pediatric skull base and the nasal cavity and to adjust exposure based on this. Radioanatomical measurements will further help tailor approaches based on developmental stage, individual anatomical characteristics, and pathology.
1.3 Brief Anatomy of the Skull Base
Skull base anatomy can be significantly distorted in children harboring skull base lesions given the deleterious effects of these lesions on the developmental process. A brief description of normal skull base anatomy is provided here for reference. The skull base is made up of several distinct bones and is comprised of the intracranial surface, subdivided into three distinct parts, and the exocranial surface. The intracranial surface has been traditionally divided into three fossae: the anterior, middle, and posterior fossa, each made up of individual bones and each with fairly distinct borders in adults. The frontal lobes rest in the anterior cranial fossa, made up of the sphenoid and ethmoid bones, paired bones that are formed after fusion of the metopic suture. An important bony structure for EEA at this level is the cribriform plate of the ethmoid bone that connects the nasal cavity and the intracranial cavity through a perforated bone traversed by olfactory nerve roots. The crista galli is an anterior bony prominence of the ethmoid bone that serves as an anchor point for the falx. The posterior border of the anterior cranial fossa is located at the level of the lesser wing of the sphenoid sinus.
The middle fossa forms through fusion of the squamous temporal bone and the parietal bone. The sella turcica is located in the center of the middle cranial fossa and harbors the pituitary gland. Immediately inferior to the sella turcica is the sphenoid sinus. The cavernous sinus, with its important anatomical structures, the carotid syphon of the internal carotids, and cranial nerves III, IV, V2, and VI, flanks the sella turcica. Neurovascular structures pass from the endocranium to the exocranium through distinct foramina. The superior orbital fissure allows passage for the oculomotor nerve, the trochlear nerve, the lacrimal, frontal and nasociliary branches of the ophthalmic nerve (V1), the abducens nerve, the superior and inferior divisions of the ophthalmic vein, and sympathetic fibers from the cavernous plexus. The maxillary nerve (V2) traverses the foramen rotundum, while the mandibular nerve (V3) traverses the foramen ovale.
The clivus is another important anatomical structure for EEA. The posterior border of the sella, along with the posterior aspect of the sphenoid bone and the basilar aspect of the occipital bone, forms the clivus. The abducens nerve runs along this sloped structure in its course toward the cavernous sinus. The posterior fossa is the largest cranial fossa and is delineated by the temporal and occipital bones laterally, as well as by the parietal bones posteriorly. The foramen magnum is located at this level and is a major passage point for the cervical spinal cord as well as vertebral arteries, anterior and posterior spinal arteries, and accessory nerve. The internal acoustic canal, part of the petrous part of the temporal bone, is also located at the level of the posterior fossa containing the facial and vestibular nerve. The jugular foramen, the third important passage point at this level, contains the glossopharyngeal nerve, the vagus nerve, and the accessory nerve along with the internal jugular vein.
The exocranial side of the skull base contains the palatine process, the zygomatic process, the palatine bone, the body of the sphenoid sinus, the petrous bones, the basioccipital bones, and the occipital condyles. An important anatomical structure of the exocranium in pediatric EEA is the pterygopalatine fossa, a cone-shaped structure in the infratemporal fossa posterior to the maxilla that is flanked by the pterygoid processes. It contains the pterygomaxillary fissure and communicates with the nasal cavity, the infratemporal fossa, the orbit, and the middle cranial fossa through different foramina: foramen rotundum, pterygoid canal, inferior orbital fissure, sphenopalatine foramen, pterygomaxillary fissure, and greater palatine canal. It contains the pterygopalatine ganglion, the terminal third of the maxillary artery, and the maxillary nerve.
1.4 Embryology of the Skull Base
The skull base undergoes a complex and highly intricate evolutionary process starting at approximately 4 weeks in utero and spanning the entire childhood. Skull base development, both in utero and postpartum, will directly impact development of the craniofacial region and the cerebrum. During this process, especially during early childhood, the cranial base is constantly being molded from above by the developing cranial vault and from below through progressive aeration of the paranasal sinuses (▶ Fig. 1.1). The cranium undergoes three distinct developmental stages: the membranous stage, the cartilaginous stage, and the ossification stage. The skull base, as well as the rostral portion of the neurocranium, originates from the rostral population of neural crest cells. At the level of the cranial vault, the coronal suture is the landmark between the structures of neural crest origin and those originating from cranial mesoderm. The developmental process is regulated by the interplay of several genes and transcription factors such as Indian hedgehog (Ihh), Sonic hedgehog (Shh), dickkopf family (Dkk1–3), Notch, Smad, Fgf, Msx, Wnt, and matrix metalloproteinases (Mmp9). Importantly, ossification of mesodermal structures occurs after formation of neurovascular elements traversing the cranial base.

Neural crest cells migrate to the embryonal pharynx before formation of the neural tube to form the embryonic arches, a mixture of ectoderm, endoderm, and mesenchymal mesodermal tissue. The cranium originates from the first two embryonic arches undergoing a gradual chondrification process, which starts at the level of the occipital bone. The anterior part of the skull base originates from neural crest cells, while the posterior skull base has mesodermal origin. The neurocranium and facial bones undergo intramembranous ossification, while the skull base undergoes endochondral ossification. The ossification process begins during week 5 of embryologic development. The notochord plays an important part, acting as the starting point of skull base development. During week 7, the cranial notochord located in the vicinity of the sella turcica induces the chondrification process of adjacent mesenchymal tissue with formation of the basioccipital bones and foramen magnum. This is followed by an ossification process. Subsequently, skull base components around the occipital bone undergo a similar chondrification and ossification process.
The rostral tip of the notochord also initiates development of the sella turcica and the anterior skull base elements. Hypophysial cartilages flanking the hypophysial pouch merge and form the primordium around the hypophysial stalk. The adenohypophysial pouch remains connected to the roof of the stomodeum, the primordial oral cavity, until the chondrification process is complete, with formation of the sella turcica. The body of the sphenoid bone forms by ossification of the presphenoid cartilages, the last portion of the median cranial base structures to undergo ossification. Thus, by the end of the third month in utero, the sphenoid region is connected with the cartilaginous nasal capsule, with almost complete formation of the cranial base. Several temporary passageways, such as the foramen cecum, the prenasal space, or the fonticulus frontalis, allow transit of other developing structures, such as dura, and normally close toward the end of the developmental process in utero.
The cartilaginous nasal capsule undergoes a similar ossification process during the fifth month of intrauterine life. This process leads to formation of the ethmoid bone, the ethmoidal labyrinth, the orbital floor, and the inferior concha. Several structures originating from the nasal capsule undergo intramembranous ossification with formation of the vomer and nasal bones, while several other portions do not undergo ossification and form the nasal septum. Similarly, mesenchymal condensation at the level of the otic capsule leads to formation of the cochlea and semicircular canals. Ossification of cartilaginous structures at these levels leads to formation of the internal acoustic meatus and the carotid canals.
1.5 Pneumatization of the Paranasal Sinuses: The Main Driving Force of Skull Base Development
The paranasal sinuses define endoscopic corridors. Aeration of the paranasal sinuses is a gradual process. Full aeration of the sinus is generally preferred prior to accessing the respective corridor. Moreover, this aeration process occurs simultaneously but in asymmetric fashion between the three main sinuses, the maxillary, ethmoid, and sphenoid sinus. The aeration process can be assessed by CT studies. More recently, thin-cut MRI scans have also been used to track the developmental process. The level of aeration will directly impact the drilling distance but will also affect neurovascular structures and restriction sites. Ultimately, safe drilling can be achieved through the soft immature bone regardless of pneumatization level especially when accessing lesions in the midline. 15 Thus, we consider pneumatization type to be a relative age-dependent limitation for EEA in children.
The sphenoid sinus is initially filled with red bone marrow. Between the ages of 7 months and 2 years, the red bone marrow is converted to yellow marrow, initially in the presphenoid plate and then extending posteriorly. 16 Effective pneumatization of the sphenoid sinus starts at 2 years and spans the entire childhood. It is thought of as the primary driving force of skull base development in children (▶ Fig. 1.1). 16 , 17 The progressive aeration of the sphenoid sinus directly impacts the transsphenoidal corridor. Certain parts of the corridor open up earlier than others and these anatomical differences can directly impact the endoscopic approach to the sinus. CT-based studies have shown that the aeration process follows a characteristic pattern, in inferior-to-superior and medial-to-lateral direction. 17 Volumetric studies using thin MRI cuts have shown that aeration occurs at a relatively slow pace. 1 Air first enters the sinus in a very exact location, namely the medial anteroinferior portion, and then expands laterally, inducing changes in both sphenoid and sellar width (▶ Fig. 1.2 and ▶ Fig. 1.3a). Access to the parasellar region is therefore optimal in older age groups. Significant volumetric and width changes at the sphenoid-sellar level occur slowly, around 11 to 13 years of age (▶ Fig. 1.2). There are no gender-dependent differences in sellar width or sinus volume. However, at the peak of the pneumatization process, higher variability in sphenoid sinus volume is also noted, due to changes in sinus width rather than length or height (▶ Fig. 1.2). The sphenoid sinus has been shown to predominantly expand in width during the aeration process, with little differences in drilling distances across age groups. Based on these findings, we advocate for separation of pediatric surgical candidates in two distinct groups. Children 11 years or younger may require more drilling and, more importantly, have limited access to laterally extending lesions through the endonasal route. After the age of 12 years, air has sufficiently penetrated the lateral-most aspects of the sphenoid sinus, rendering access to the parasellar area easier and safer (▶ Fig. 1.3). Nonetheless, the pneumatization process can be frequently incomplete, with approximately 13 to 25% of adolescents and adults having a presellar pneumatization pattern at the end of the developmental process. 17 In such cases, air is solely localized in front of the sella turcica, requiring a more anterior entry point for transsphenoidal EEA.


The ethmoid sinus has a different pneumatization pattern, with air cells present immediately after birth at the anterior-most aspect of the sinus. 16 Air progressively penetrates posteriorly until puberty, for approximately 8 to 12 years, such that the medial and lateral walls of the sinus become parallel in anterior-to-posterior direction. The medial and inferior portions of the sinus are the last ones to become fully pneumatized, through extension of the anterior ethmoidal air cells. This inferomedial pneumatization process can also start at the level of the middle ethmoidal cells, creating a normal variant, the concha bullosa. The pneumatization process can also progress in an inferolateral direction, with extension along the roof of the maxillary sinus and the inferior lamina papyracea. During the normal pneumatization process, ethmoidal air can also be located at the level of the maxillary sinus ostium. Importantly, while the size of the ethmoid air cells changes with aeration, the relationship with the meatus does not change in evolution. During transethmoidal approaches, the pneumatization level and pattern of the ethmoid sinus can aid in adequate planning of the most appropriate surgical trajectory.
The maxillary sinus also undergoes a progressive aeration process, which will change its anatomical relationships. The rudimentary sinus has an initial volume of approximately 8 cm3 and is located under the medial orbital wall. As it undergoes pneumatization, the sinus extends in lateral and inferior direction and reaches the maxillary bone and hard palate at approximately 9 years. 16 The final phases of the aeration process occur after the eruption of permanent teeth and push the floor of the sinus below the floor of the nasal cavity.
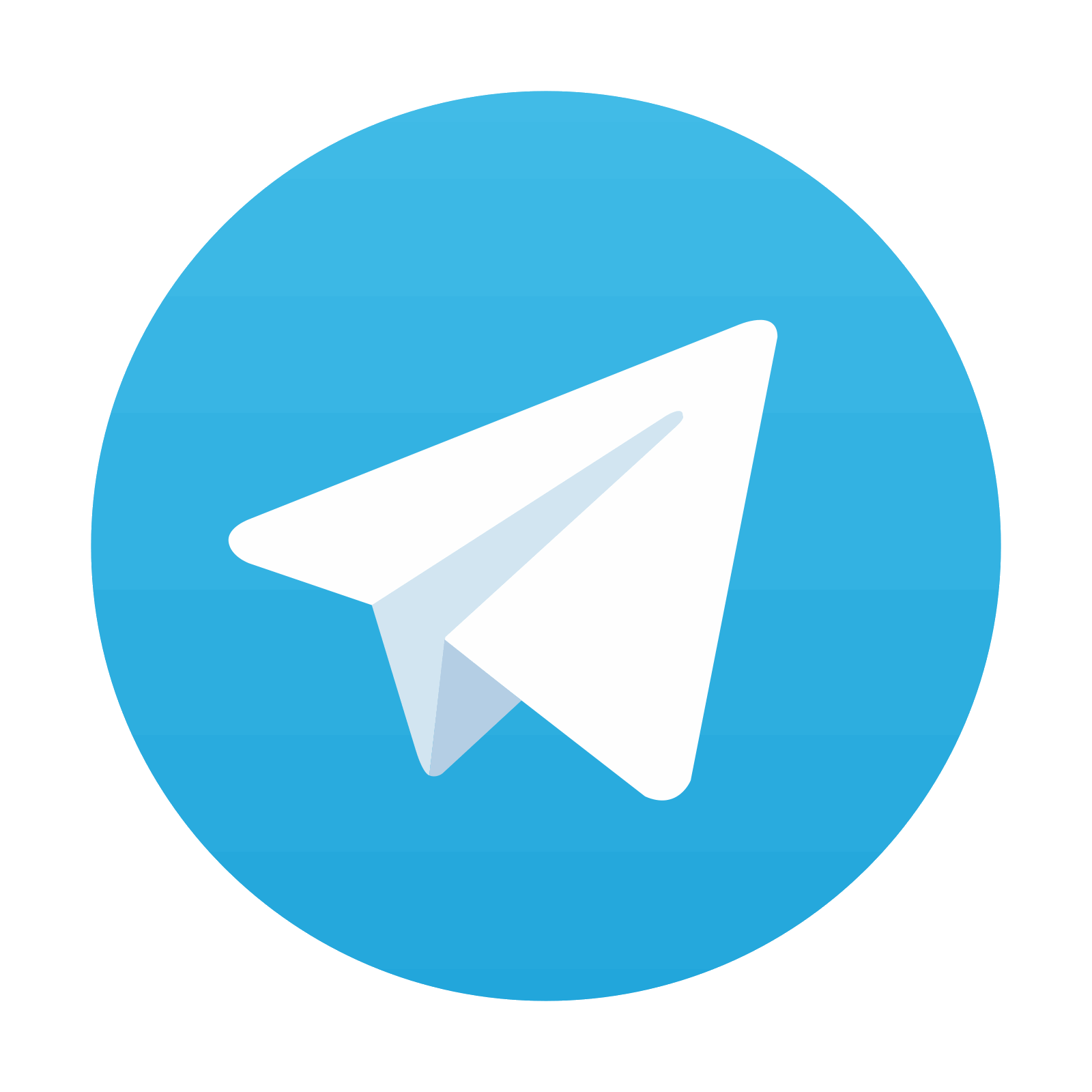
Stay updated, free articles. Join our Telegram channel
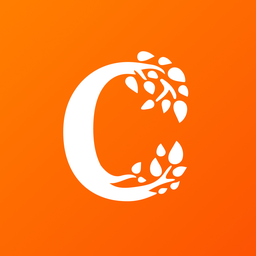
Full access? Get Clinical Tree
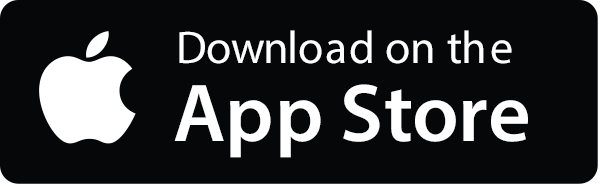
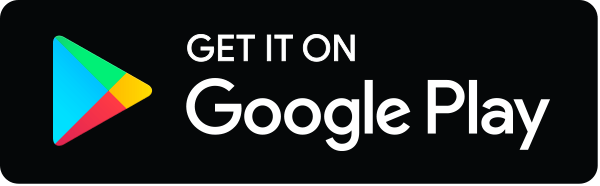