1 Biomechanically Relevant Anatomy and Material Properties of the Spine and Associated Elements
1.1 Anatomy
The vertebral column complex consists of ventrally located vertebral bodies and intervening intervertebral discs that collectively assume most of the axial load-bearing responsibilities of the spine.1 The pedicles connect the ventral and the dorsal components of each spinal segment. The laminae provide a roof for the spinal canal, whereas the facet joints limit rotation, flexion, extension, lateral bending, and translation to varying extents—depending on the region. The muscles and ligaments provide for, and also limit, torso movement. In addition, they contribute to axial load bearing.
Many of the figures in this chapter reflect the sum of information gleaned from a number of sources. Occasionally, the data from these sources vary widely. Average data, therefore, are presented in a figure format in order to convey general values and trends. Because of gaps in the available information, some figures depict extrapolated data when appropriate.
1.1.1 The Vertebral Body
Both the width and depth of the vertebral bodies increase as the spine is descended in a rostral-to-caudal direction (Fig. 1.1).2–5 The vertebral body height also increases in a rostral-to-caudal direction, with the exception of a slight reversal of this relationship at the C6 and lower lumbar levels (Fig. 1.2).2–5 The height of the C6 vertebral body is usually less than the heights of the C5 and C7 bodies, and the heights of the lower lumbar vertebral bodies are usually less than the height of the L2 vertebral body. In the cervical spine, the uncinate process projects from the rostral–dorsal–lateral aspect of each vertebral body (C3 through C7) (Fig. 1.3). It participates in stabilization of the motion segment during axial rotation and form the nidus from which foraminal osteophytes can emerge.6 The uncovertebral joint allows an articulation of this process with the caudal–dorsal–lateral aspect of the vertebral body above. This is essentially an extension of the intervertebral disc that plays a role in the coupling phenomenon (see Chapter 2) and in both the facilitation and limitation of rotation.7



The progressive increase in size of the vertebral bodies, observed as one descends the spine, correlates with strength and load-bearing ability. A lesser incidence of spine fractures observed in the lower lumbar spine, compared to more rostral regions, is related, at least in part, to the size and increased strength of the vertebrae in this region. This correlates with vertebral body volume8 and the axial load–resisting ability of the spine (Fig. 1.4).5,9–13 Of note, the vertebral endplates which abut the disc space rostrally and caudally, resist compression from the intervertebral disc and thus contribute to the provision of axial load–bearing ability. There exist variable location-specific endplate strength and stiffness characteristics. For example, the center of the lumbar endplate is the weakest and the perimeter is the strongest regarding axial load–bearing ability and stiffness. This has significant implications regarding the location of interbody spacer placement (see Chapters 15 and 21).

The shape of the vertebral body varies from region to region. Although its shape is generally consistent with that of a solid cylinder, the dorsal aspect of the vertebral body (the surface facing the spinal canal) is concave dorsally (Fig. 1.5). This is particularly significant in ventral spinal operations in which screw purchase of the dorsal vertebral body cortex is critical. Misinterpretation of the lateral radiograph may lead to neural impingement by the screw.
The costovertebral joint contributes to spinal stability by providing an articulation to the rib. This is particularly true with respect to lateral bending and axial rotation.

1.1.2 The Facet Joints
The facet joints do not, in and of themselves, substantially support axial loads unless the spine is in an extension posture (lordosis). They are apophyseal joints that have a loose capsule and a synovial lining. These joints are also known as diarthrodial or synovial joints. In the cervical spine, the facet joints are primarily oriented in a coronal plane (Fig. 1.6a).5 The orientation of the facet joints changes significantly as one descends the thoracic and lumbar spine (Fig. 1.6b, c). The angle (from midline) increases from L1 to L5 (Fig. 1.6d).

The facet joint surfaces of C3 through C7 face the instantaneous axis of rotation (IAR; the axis about which a vertebral segment rotates) and are not particularly restrictive of gliding movements (e.g., rotation and bending). The ability of the cervical spine facet joints to resist flexion–extension, lateral bending, and rotation are relatively diminished because of this coronal plane orientation. Hence, such movement is substantial in this region (Fig. 1.7).5,14,15 The cervical facet joint anatomy should be carefully scrutinized before lateral cervical mass screw placement. Otherwise, joint violation by the screw may be an undesirable result, particularly at the caudal joint.16

In the lumbar region, the facet joints are oriented in the sagittal plane (see Fig. 1.6).5,17,18 Their ability to resist flexion or translational movement in this region is minimal, whereas their ability to resist rotation is substantial (see Fig. 1.7).
The nearly coronal facet orientation at L5–S1 is a factor in the relatively decreased incidence of subluxation, in the presence of intact facet joints, at the lumbosacral joint; that is, in degenerative spondylolisthesis, subluxation is more common at L4–L5 than at L5–S1 despite the relative vertical orientation of the L5–S1 disc interspace.
The facet joints bear a greater fraction of the axial load if the spine is oriented in extension (i.e., in the cervical and lumbar regions). This obviously varies with the type of load.19
1.1.3 The Lamina, Spinal Canal, and Spinal Canal Contents
The lamina provides dorsal protection for the dural sac and a foundation for the spinous processes, which serve as solid attachment sites for muscles and ligaments. This is enhanced by its anatomical characteristics.20 Forces applied via the spinous processes cause movement of the spine. The dura mater provides a tough covering over the spinal cord and axial neural elements. It is innervated by an extensive distribution of nerve fibers, as are the longitudinal ligaments.21
The tracts within the spinal cord in the cervical and thoracic regions and the nerve roots within the lumbar region are somatotopically oriented. This orientation is consistent. In the region of the spinal cord, the corticospinal tracts are somatotopically arranged so that the hand function is located most medially and foot function is located laterally. The spinothalamic tract is arranged so that hand sensation is located medially and ventrally, whereas sacral sensation is located dorsally and laterally. The posterior columns are similarly arranged in a somatotopic manner. In the lumbar region, the nerve roots are arranged so that the lower sacral segments are located medially and the exiting upper lumbar segments are oriented laterally (Fig. 1.8).22

The spinal canal dimensions, and hence the extramedullary space in the nonpathologic spine, are generous in youth and maturity.23 In the upper cervical region, they are the most generous, and in the upper thoracic region, the least so. In the lumbar region, both the epidural and intradural space are, in general, capacious (Fig. 1.9 and Fig. 1.10).2–4,24 In the case of a preexisting spinal stenosis, however, the factor of safety may be small. This is important when the surgeon is considering a spinal instrumentation application that might impinge upon the neural elements. Subluxation, as an aside, has a predictable effect on spinal canal encroachment.25


The depth of the lumbar spinal canal does not change significantly as one descends from the upper to the lower lumbar regions, but its width increases (see Fig. 1.9). The cross-sectional area of the lumbar and sacral canal is generous. It contains the cauda equina, which is relatively resistant to neurologic insults (compared with the spinal cord proper). Therefore, posttraumatic lumbar neural element injury in the lumbar region is less common than injury associated with comparable spinal column deformation elsewhere in the spine.
The shape of the spinal canal itself varies along its length. In the cervical, thoracic, and upper lumbar regions, the shape of the spinal canal is one of a “ballooned” triangle. Toward the lumbosacral junction, however, it assumes a bicorne configuration resembling “Napoleon’s hat” (see Fig. 1.10).17,26
1.1.4 The Pedicle
A sound knowledge of pedicle anatomy is critical in many surgical scenarios.7,26–30 The pedicles of the cervical spine are shorter and proportionally of greater diameter than those in other regions of the spine.7,27 The transverse pedicle width gradually decreases from the cervical to the midthoracic region and then increases as one descends the lumbar spine (Fig. 1.11).3,31,32 The pedicle height (sagittal pedicle width) increases gradually (except at C2) from the cervical to the thoracolumbar region and then decreases as one descends the lumbar spine (Fig. 1.12).3,31,32 This relationship is favorable for transpedicular screw placement in the lumbar spine because pedicle width is more important than height in this regard. A small variation in pedicle height (sagittal pedicle width) in the lumbar region is not clinically significant because of the already generous dimension (see Fig. 1.12).31,32


The transverse pedicle angle decreases from the cervical spine to the thoracolumbar region and then increases as the lumbar spine is descended (Fig. 1.13).3,31–33 This necessitates a wider angle of approach for the placement of pedicle screws in the low lumbar spine. An appreciation of vertebral anatomy is similarly important when pedicle screws are to be placed in the sacral region.34,35 There is, however, usually a greater margin of safety with regard to screw placement.

Also important, particularly regarding pedicle screw placement in the upper lumbar and thoracic spine (where the margin of safety is less than in the low lumbar region), is the sagittal pedicle angle (Fig. 1.14)3,31,32 and the relationship of adjacent neural structures.36,37 In the upper lumbar and thoracic spine, the sagittal pedicle angle becomes relatively steep.

McCormack and colleagues described the unique relationship between the thoracic pedicle and adjacent transverse process.38 They, in fact, objectively portrayed this with the following equation:
Equation s. Kap.
where D= the rostrocaudal distance from the midpoint of the transverse process to the midpoint of the pedicle and TL = thoracic level. This relationship is illustrated in Fig. 1.15. This may provide assistance to the surgeon during the placement of thoracic pedicle screws. Of note is that pedicle morphology is relatively unaffected by race39 but significantly affected by age (youth).40

Pedicle strength, particularly cortical strength, characteristics are of substantial relevance. The technique of screw insertion, for example, affects the deformation behavior of the pedicle cortex.41 This is related, in part, to the microstructure morphology.42 These factors should affect surgeon considerations for the selection of pedicle screws and the location of their placement.
1.1.5 The Intervertebral Disc
The intervertebral discs are composed of a nucleus pulposus and an annulus fibrosus, which provide support, absorb shock, and both allow and resist some excessive movement. The discs have no synovial fluid or synovial lining. They are classified as amphiarthrodial joints. Their ability to resist axial loads is substantial,43 but it decreases with age.18 In the thoracic spine, axial load–bearing ability is increased by the costovertebral joint, which supplements the strength of the intervertebral disc.44 The addition of flexion, extension, or lateral-bending force vectors, however, causes significant deformity of the disc interspace and fosters disc bulging and herniation. The disc itself is surrounded by an endplate that resists herniation of the disc into the vertebral body (Schmorl’s node) and that has a predictable shape.45
The annulus fibrosus is composed of several layers of radiating fibers attached to the cartilaginous endplates (inner fibers) and the cortical bone on the walls of the vertebral body (Sharpey’s fibers). In the cervical region, the annulus is more like a crescent-shaped anterior interosseous ligament.46 These components incompletely resist deformation (Fig. 1.16). Because of their angled orientation (approximately 30 degrees with respect to the endplate), the annulus fibrosus fibers resist rotation with relative effectiveness. However, they do not resist compression as well. Note that disc bulging occurs on the concave side of a bending spine (Fig. 1.16c). This correlates with osteophyte formation. Disc bulging, however, should not be confused with disc herniation. The former is caused by distortion of the annulus fibrosus and is associated with eccentric loading. The latter is caused by migration of the nucleus pulposus from its normal anatomical location.
In contrast to the direction of disc (annulus) bulging (i.e., toward the concavity of a spinal bend), the nucleus pulposus moves in the opposite direction (Fig. 1.16d).47 Flexion therefore causes bulging of the annulus fibrosus ventrally. It also causes a tendency of the nucleus pulposus to migrate dorsally. Of note, the annulus is exposed to significant strain during normal physiologic loading.48

1.1.6 The Transverse Process
The transverse processes provide a site for attachment of the paraspinous muscles. As moment arms for attached muscles increase, they provide increased leverage for lateral bending. They, hence, are easily fractured because of their relatively small size and poor vascularization. This is particularly so in the lumbar region, where applied loads are often substantial.
The transverse processes arise from the junction of the pedicle and the lamina. In the middle to lower thoracic region, they are reasonably substantial and project in a lateral and slightly upward direction. Their projection from the spine is roughly at the same anteroposterior plane as the facet joints and dorsal aspect of the pedicles. In the lower thoracic region, the transverse processes become increasingly smaller and thus are less useful for hook placement. In the thoracic spine, a unique level-dependent relationship between the pedicle and the transverse process exists, as defined by McCormack and colleagues (see previous section “The Pedicle” in this chapter).38
In the lumbar region, the transverse processes project from the spine in a more ventral and anteroposterior position. They become more substantial and thus are able to become sites for bony fusion. Their utility for this purpose, however, is limited by their relatively poor blood supply and their often less-than-optimal robustness.
In the upper six cervical vertebrae, the vertebral artery is usually transmitted through the foramen transversarium. Of note is that the foramen transversarium is juxtaposed to the uncovertebral joint.
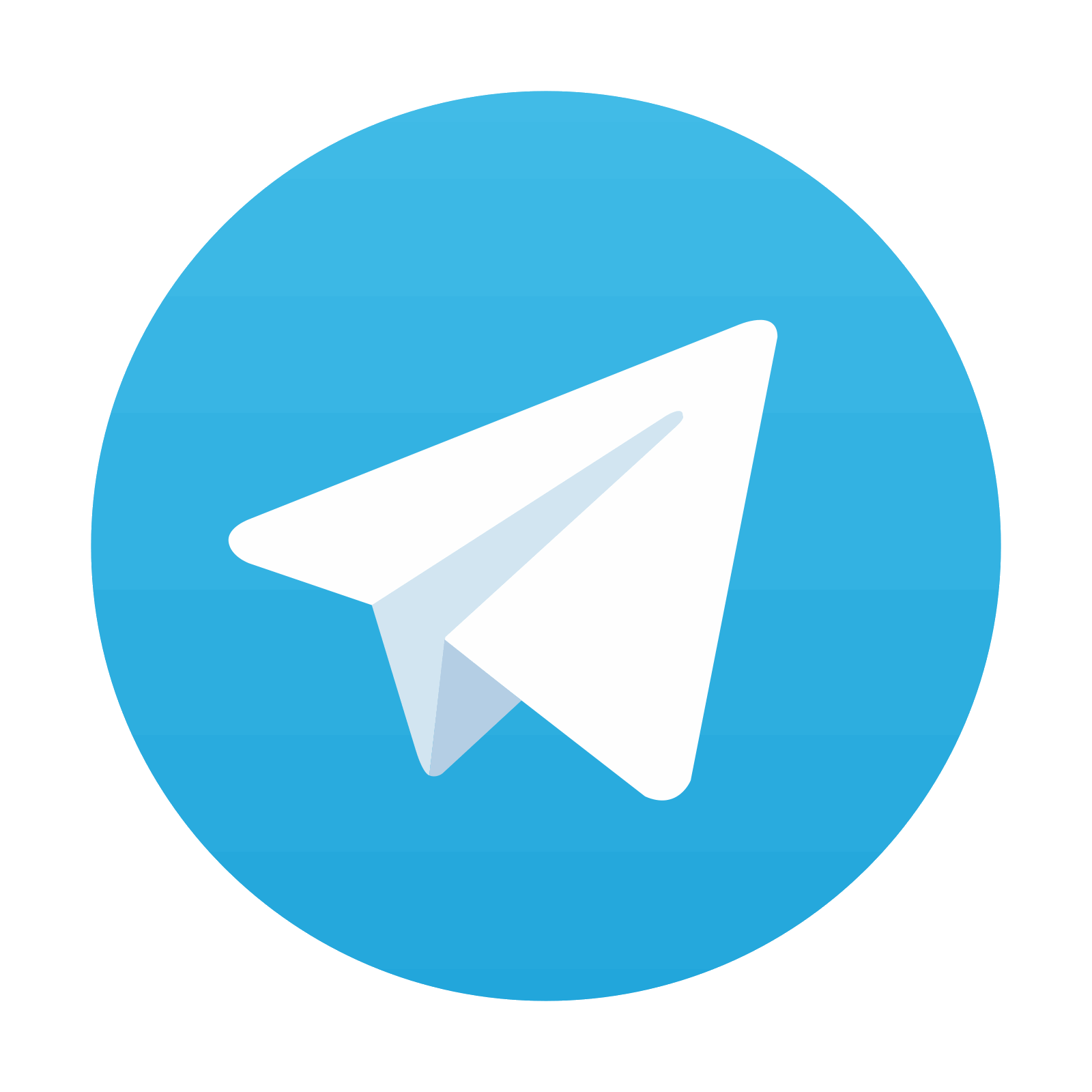
Stay updated, free articles. Join our Telegram channel
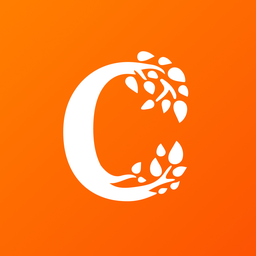
Full access? Get Clinical Tree
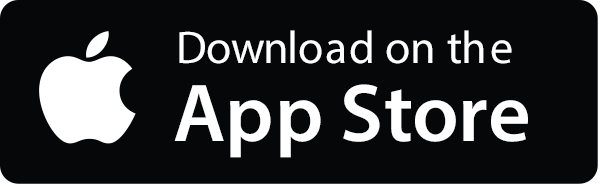
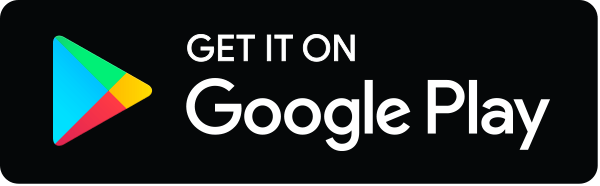
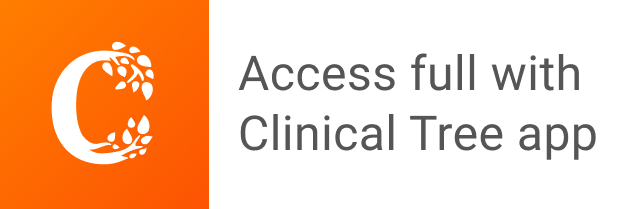