12 Motor Mapping (Rolandic, Pre-Rolandic, and Insular Cortex)
Abstract
This chapter discusses brain parenchymal motor mapping in the perioperative period with glioma as the representative type of tumor. We discuss multimodal techniques for preoperative motor function localization such as comprehensive neuropsychological evaluation and functional brain imaging; neurophysiological methods for intraoperative motor cortex and subcortical motor pathway localization and monitoring; motor mapping-related neuroanesthesia techniques, and advantages and disadvantages of asleep and awake intraoperative mapping; and a typical case illustrating motor mapping.
12.1 Introduction
The extent of resection of gliomas has been shown to be most important in the treatment of low- and high-grade gliomas. Since gliomas infiltrate beyond boundaries shown by magnetic resonance imaging (MRI), it has been proposed that true tumor resection encompasses excision beyond tumor boundaries shown by MRI. By excising beyond the traditional fluid-attenuated inversion recovery “true” lesion range, cancer progression is delayed. After defining the benefits of surgery, the primary objective of glioma surgery at present is maximal safe tumor resection to reduce tumor cell burden, thus improving the response rate and efficacy of subsequent adjuvant therapy. In our division, we studied more than 800 patients who underwent surgery for glioma with or without intraoperative neurophysiological monitoring (IONM). Direct electrical stimulation of the brain in functional areas was found to not only reduce the severity of nerve injuries, but also expand the extent of the resection. 1 This explains why intraoperative brain mapping has become the “golden standard” for tumor resection in functional areas of brain. Depending on individualized cortical and subcortical functional boundaries, true maximum safe resection is aimed with the goal of providing patients with balanced oncological and functional benefits.
The motor cortex is located anterior to the central sulcus (rolandic fissure) and can be divided into the rolandic (primary motor and sensory) cortex, pre-rolandic cortex (premotor cortex), and the supplementary motor area (SMA; Fig. 12‑1). The rolandic cortex, also called the central lobe, consists of the precentral and postcentral gyri. 2 Primary motor cortex is the Brodmann area 4 that consists of most parts of precentral gyrus and the anterior part of paracentral lobule in the medial aspect of frontal lobe. Premotor cortex generally corresponds to Brodmann area 6 and consists of inferior portion of precentral gyrus, posterior parts of superior and middle frontal gyri, and part of medial aspect of superior frontal gyrus. The pre-rolandic cortex and SMA comprise the secondary motor cortex. The insular cortex lies deep within the lateral sulcus like an island and is covered by the insular opercula and is surrounded by critical neural pathways.

12.2 Preoperative Brain Function Localization
12.2.1 Neuropsychological Assessment
In the preoperative work-up of glioma patients, neuropsychological evaluation is very important. The commonly used neuropsychological assessment scales are Edinburgh Handedness Inventory, Karnofsky Performance Score (KPS), Mini Mental State Examination (MMSE), Boston Naming Test (BNT), and the Aphasia Battery test. All patients must undergo neuropsychological assessment before and after surgery, since a comprehensive understanding of all types of dominant or recessive neurological dysfunctions before surgery allows surgeons to provide individualized treatment, predict possible temporary postoperative deficits, develop postoperative rehabilitation program, and provide a baseline for continuous assessment of postoperative neurological function.
12.2.2 Functional Magnetic Resonance Imaging
Functional MRI (fMRI) is the most common functional neuroimaging technique. When neurons are excited, the neurovascular coupling mechanism leads to a local increase in the ratio of oxyhemoglobin (antimagnetic) to deoxyhemoglobin (paramagnetic), which can be detected by echo-planner imaging, a fast MRI imaging technique. Although fMRI is fairly accurate in localizing primary motor 3 and sensory cortices, it is less sensitive in localizing language. 4 There are some important considerations underlying fMRI usage in brain tumor surgery. First, fMRI only shows areas of increased cortical blood flow which is an indirect measure of neuronal activity, and is susceptible to errors in the peritumoral brain. Furthermore, there is a temporal delay between neuronal activity and changes in blood flow; therefore, the transient activation of brain regions cannot be detected. In essence, fMRI best reveals areas of the gray matter involved in a particular task but does not indicate areas that are functionally necessary. Therefore, relying on fMRI 3 , 4 to guide surgery may result in excision of critical functional cortical regions (false negative) or hinder neurosurgeons from removing noncritical cortical regions (false positive) that patients can actually compensate for. More appropriate uses of fMRI include 1 preoperative surgical planning and rehearsal 2 ; screening for functional brain regions that need to be verified by intraoperative direct electrical stimulation; or 3 providing cortical motor network information, such as SMA, for patients unable to undergo awake craniotomy.
12.2.3 Transcranial Magnetic Stimulation
This is a newer noninvasive preoperative cortical function mapping technique that involves altering the action potential of cortical neurons through a time-varying magnetic field and combines with navigation technology to accurately localize functional cortical areas. Recent studies have shown that transcranial magnetic stimulation (TMS) localization of cortical motor areas concurs with results of intraoperative direct electrical stimulation. 5 , 6 TMS can be used in healthy subjects and patients with brain lesions. It can also be used to study cortical function remodeling due to its ability of regulating cortical excitability.
12.2.4 Diffusion Tensor Imaging
This technique is based on anisotropic imaging of the diffusion of water molecules in white matter fibers and can be used to form three-dimensional models of subcortical neural conduction pathways. Tracking imaging (tractography) shows the morphology, structure, and projection of these pathways. In addition to diffusion tensor imaging (DTI) tractography being important for research and teaching, it provides information about virtual structure rather than real function. Brain tumors are often accompanied by edema resulting in distortion and erosion of DTI white matter tracts. Results from our division’s clinical trial demonstrated that DTI tractography is clinically effective but not completely reliable in delineating descending motor pathways (corticospinal tract) and must be integrated with direct cortical stimulation for functional glioma surgery (Fig. 12‑2). 7 Despite the utility of fMRI and DTI in localizing functional cortical areas and subcortical neural pathways, respectively, a multimodal imaging approach comprising IONM is essential to ensure the accuracy and reliability of intraoperative functional brain mapping.

12.3 Intraoperative Neurophysiological Monitoring
Preoperatively, anesthesiologists, electrophysiologists, and surgeons discuss the anesthesia method, monitoring mode, and monitoring parameters for intraoperative cortical and subcortical neural pathways according to the surgical plan. IONM comprises four parts: somatosensory evoked potentials (SSEP) to locate the central sulcus, motor evoked potentials (MEP) to monitor motor pathway integrity, direct cortical electrical stimulation (DCS) in combination with electromyography (EMG) recording to map the primary motor cortex, and direct subcortical electrical stimulation (DsCS) to delineate the subcortical motor pathway (corticospinal tract). In a recent study, we divided primary glioma patients into two groups based on whether or not IONM was applied intraoperatively. The proportion of tumors located in the dominant hemisphere and the eloquent cortex was higher in the IONM versus the non-IONM group. We found the rate of postoperative hospital stay, long-term language deficit, and overall neurological dysfunction to be significantly lower in the IONM group compared to the non-IONM group, suggesting that IONM could reduce the rate of postoperative neurological dysfunction. 1 However, questions still remain on how IONM precisely influences extent of resection in glioma surgery and overall survival.
12.3.1 Somatosensory Evoked Potential
SSEP involves the stimulation of action potentials in peripheral nerves that are recorded in the cerebral cortex which, to some extent, reflect the afferent pathways of specific somatic sensations, the reticular structure of the brainstem, and the functional state of the cerebral cortex. SSEP recording is characterized by continuity, repeatability, and identifiable waveforms, and is advantageous in terms of anatomical monitoring and technique. The stimulation technique involves repetitive stimuli of intensity 15 to 25 mA and duration 0.10 to 0.30 ms at a rate of 2.10 to 4.70 Hz.
An important initial step in sensorimotor function mapping is identifying the central sulcus by SSEP phase reversal technique (Fig. 12‑3). A strip electrode with multiple contacts is placed perpendicularly on the surface of the presumed central sulcus to record SSEP by stimulating the median nerve (Fig. 12‑4). The strip electrode is moved until it demonstrates polarity reversal. 8 From this, the surgeon and neurophysiologist can estimate the position of the central sulcus which lies between the contacts where polarity reversal occurs. Although it is possible to identify the central sulcus by analyzing anatomical landmarks on neuroimaging, 9 anatomical and neurophysiological discrepancies resulting from large rolandic and pre-rolandic brain lesions can significantly impact the reliability of direct visual identification of the central sulcus.


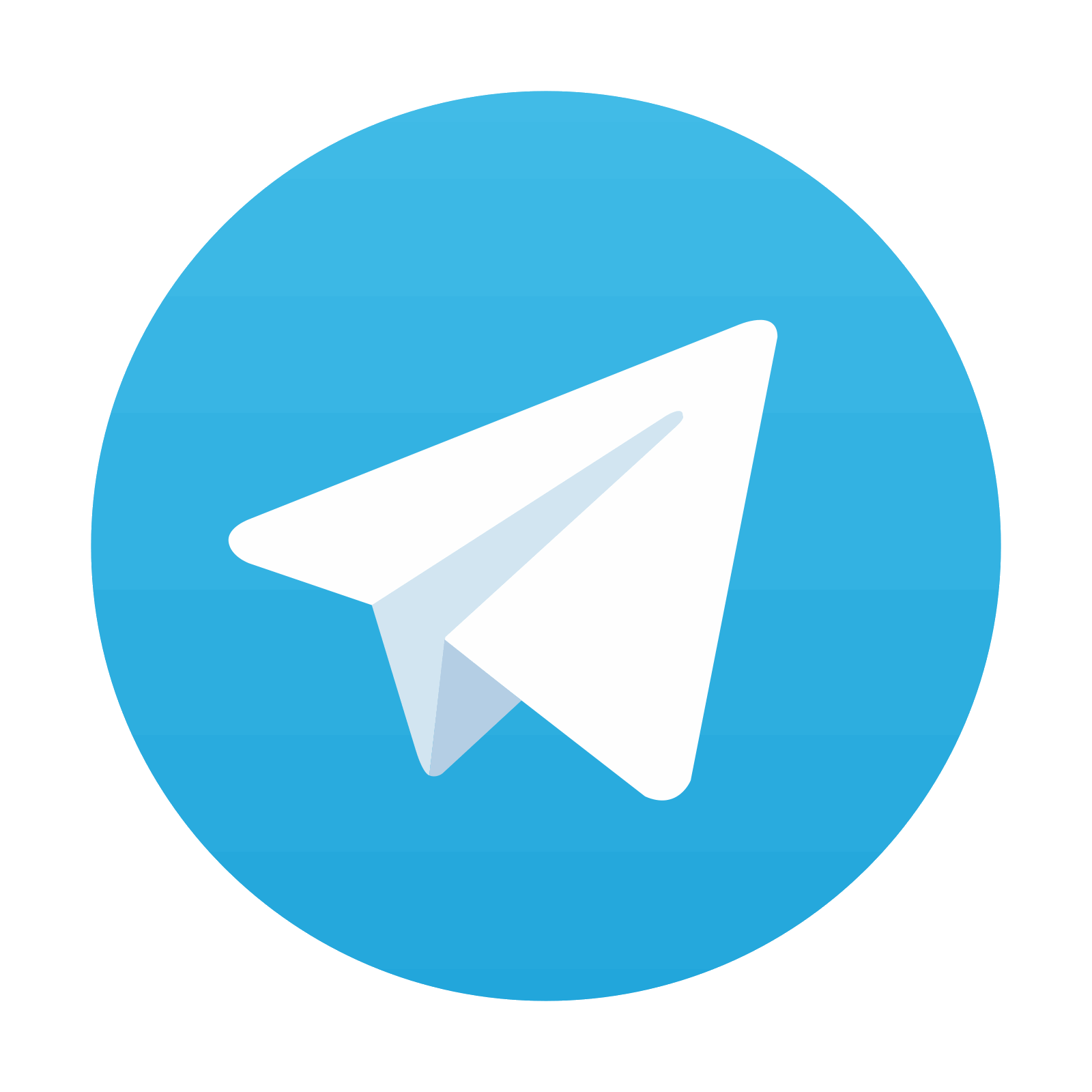
Stay updated, free articles. Join our Telegram channel
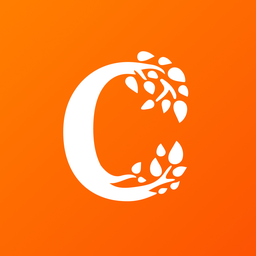
Full access? Get Clinical Tree
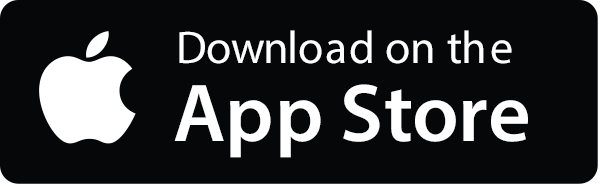
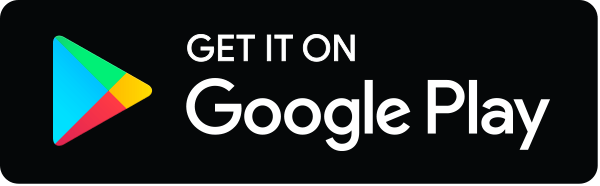
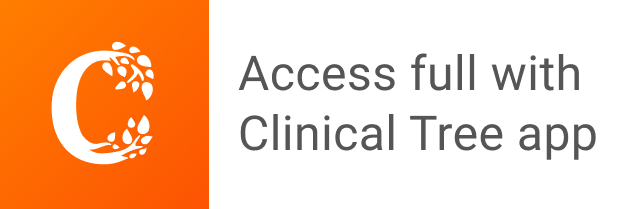