13 Implant Material Properties
13.1 Metals
13.1.1 Elements and Alloys
Metallurgy is the study of metals, their material properties, and their shaping and treatment by heating and/or cooling. At least a rudimentary knowledge of this discipline is important for surgeons performing reconstructive spine operations. Without this knowledge, inappropriate decisions may be made regarding implant or construct selection. This chapter introduces the metallurgical principles crucial to this complex periphery of medicine.
An element is a simple substance that cannot be separated into simpler components by routine chemical means. An alloy is made by mixing and melding together two or more metal elements, or an element and some other substance. The mixing and melding of two or more elements of appropriate atomic numbers may yield an alloy that is useful in the manufacture of spinal implants. These elements include (with their standard abbreviations and atomic numbers in parentheses) the following: aluminum (Al, 13); titanium (Ti, 22); vanadium (V, 23); chromium (Cr, 24); manganese (Mn, 25); iron (Fe, 26); cobalt (Co, 27); nickel (Ni, 28); zirconium (Zr, 40); niobium (Nb, 41); and molybdenum (Mo, 42). Titanium is the only element that is commonly used in an unalloyed (“pure”) form as an implant material.
Other elements commonly found in metals are hydrogen (H, 1); carbon (C, 6); nitrogen (N, 7); and oxygen (O, 8). These elements are essentially contaminants. However, they may stabilize certain phases of some metals when present in small amounts. For example, small amounts of carbon and nitrogen may stabilize the alpha phase of titanium. The presence of contaminants and the unavoidable difficulty of eliminating them necessitates the grading of metals such as titanium.
“Pure” (unalloyed) titanium is available in four grades. Each contains varying composition limits of multiple contaminants (including iron). Some of these contaminants are included by design; others are included because of difficulty of removal. Grade 1 is the purest and grade 4 the least pure. The strength of unalloyed titanium increases as the oxygen (a contaminant) content increases (0.18 to 0.40%).
Although the density and modulus of elasticity of unalloyed titanium do not significantly change from grade to grade, the ultimate and 0.2% tensile yield strengths of titanium depend largely on its grade.1 The modulus of elasticity (elastic modulus) of a material describes the stress (force per unit of cross-sectional area) per unit of strain (linear deformation per unit of length) in the elastic region. A higher modulus of elasticity implies a stiffer, or more rigid, implant (see Chapter 2). The ultimate and 0.2% tensile yield strengths are the highest tolerable stress (to failure) and the stress that causes a 0.2% deformation, respectively.
The purest titanium (grade 1) is less able to tolerate “stretch” than the least pure unalloyed grades—that is, the various grades have different tensile strengths. The less pure titanium (grades 2 through 4) are similar in this regard to 316L stainless steel. (All have relatively high tensile strengths.) On the other hand, 316L stainless steel is stiffer (i.e., has a higher modulus of elasticity) than all grades of unalloyed titanium. Therefore, it results in a relatively increased transfer of stress from the implant to bone. This property augments stress shielding when 316L stainless steel is used with rigid systems, such as fixed moment arm cantilever beam constructs (see Chapters 16 and 17).
Many alloys are used in the manufacture of spinal implants. These include 316L stainless steel (Cr 17%, Ni 13%, Mo 2.25%, with Fe and C), cast Co-Cr-Mo, Ti-6Al-4V (Ti with 6% Al and 4% V), and most recently, Ti with 13% Nb and 13% Zr (74% titanium). 316L stainless steel can be subjected to a diffusion-harboring treatment that creates a ceramic-like surface, which augments resistance to wear and fretting. With regard to stainless steel, chromium provides a corrosion-resistant oxide film. Molybdenum provides resistance to pitting corrosion. Nickel provides corrosion resistance, as well.2 For many reasons, stainless steel implants have fallen out of vogue. These reasons include greater risk for chronic infection, image degradation, and nickel allergy. Ti-6Al-4V has now essentially replaced 316L stainless steel for most spine implant applications.
Another alloy, Vitallium, has also found utility in spine applications. Vitallium is a trademarked alloy composed of Co and Cr. Finally, another stainless steel alloy has been introduced clinically. It is composed of 22% Cr, 13% Ni, and 5% Mn and is termed 22–13–5 stainless steel. Its modulus of elasticity in tension is similar to that of 316L stainless steel, but its ultimate tensile strength is roughly twice that of 316L stainless steel.
13.1.2 Material Properties
The term ductility describes, in a sense, the deformability of an object. A ductile object is one that can permanently deform before failure. The converse of ductility is brittleness. Brittle objects fail without permanent deformation.
Metals may be elastically deformed until the yield point is reached. Plastic deformation ensues if further force is applied until ultimate failure occurs. Conversely, bone fractures without permanent deformation. The yield point of bone is equal to its ultimate failure point (see Chapter 2).
Fatigue failure occurs because of the cumulative alterations of structure (damage) related to cyclical loading. The average spine cycles about 3 million times per year.3 Clinical implant failure rarely occurs after the application of a load that exceeds the static strength of the implant. Instead, implants usually fail after cyclical loading and implant fatigue. Of note, materials have unique responses to cyclical loading. Thus, titanium and stainless steel respond differently to cyclical loading, as well as to the rate of loading. For example, at lower frequencies of loading (4 Hz), titanium performs better than stainless steel, whereas at 16 Hz, they perform equally.4 Of note is that the normal rate of loading is approximately 0.2 Hz.
13.1.3 Surface Characteristics and Their Alteration
The surface characteristics of a spinal implant affect its performance through (1) corrosion, (2) material properties, and (3) component–component interface friction. The selection of implant material depends, in part, on all three of these characteristics.
Corrosion is the degeneration of a metal by oxidation or a related process. Corrosion, with consequent metal weakening, is a potential complication of the exposure of an implant to a foreign environment, such as biological tissues.5–9 Such rarely affects spinal stability, however. This is so because bone graft incorporation usually occurs long before corrosion-related metal failure can occur clinically. Resistance to surface corrosion increases as the anodic breakdown potential increases (Fig. 13.1).1 Anodic breakdown potential is the voltage at which the anodic formation of barrier oxide films, which resist corrosion, breaks down. A high anodic breakdown potential implies the formation of a greater protective barrier oxide film. As the iron content of an alloy is increased, the corrosion rate is increased. Corrosion resistance can be quantified by measuring the anodic polarization behavior of a specific metal against a control (e.g., a saturated calomel electrode in a physiologic [Hanks] solution). Titanium is much more resistant to corrosion than 316L stainless steel. Cast Co-Cr-Mo and Ti-6A1–4V are intermediate. Again, this protectivity against corrosion is related to titanium’s characteristic development of surface film (oxide). The surface film reforms if the metal is scratched or abraded. The surface film on titanium is both more stable and more resistant to corrosion than that on 316L stainless steel and other alloys. However, even a trace amount of iron in titanium decreases the stability of the protective film.

Occasionally, a limited extent of surface corrosion is desirable. As mentioned above, titanium forms a passive surface film that protects it against chemical attack. This type of protection may be enhanced, in certain circumstances, by a process termed anodizing. Anodizing is an electrolytic process that increases the thickness of a naturally occurring surface layer of oxide. It is used to increase stability and corrosion resistance.
Corrosion occurring within crevices and small cavities on a metal surface, usually at the junction of two similar metals, is termed crevice corrosion. Titanium is much more resistant to this process than 316L stainless steel. A very high fraction (> 70%) of retrieved 316L stainless steel plates were shown to be associated with a high incidence of corrosion, which was dependent on device design.5 Rarely, intraspinal metallosis (formation of granulation tissue adjacent to an implant) and delayed neurologic symptoms has been observed to be associated with 316L stainless steel spine instrumentation.6 Titanium particulate debris that is introduced at the level of a spine arthrodesis has been shown to elicit a cytokine-mediated particulate-induced response that may serve as the impetus for late inflammatory responses and osteolysis.7,8
A form of corrosion that can occur when the protective passive film (the surface layer of oxide) is mechanically disrupted—usually via a repetitive friction mechanism—is fretting corrosion (corrosion wear attack). This most commonly occurs at metal–metal interfaces. Titanium is much more resistant to fretting corrosion than is 316L stainless steel. Along with metallurgical factors, the type of component–construct interface plays a role in corrosion The hook–rod interface of a Harrington distraction system produces much less fretting corrosion than does the wire–rod interface of a Luque rod–wire system (both 316L stainless steel).10 Ti-6A1–4V is particularly prone to fretting corrosion, a property that somewhat limits its utility. The relatively tight interfaces between components, however, considerably reduces the importance of this factor.
Environmentally assisted cracking is another mechanism of surface failure that usually occurs at metal–metal interfaces (Fig. 13.2). A stress riser exists at such interfaces. This most certainly contributes to the process.

An accelerated form of corrosion that can occur in a mixed metal system, on account of the difference in electrochemical potential between the two metals, is galvanic corrosion. This phenomenon is usually clinically insignificant; for example, the use of titanium and stainless steel together causes no known significant clinical sequelae. Liability considerations, however, must be taken into account.
Osseointegration is the direct bonding of bone to an implant. The surface material properties dictate the osseointegration potential of any given material. Of all the materials commonly used for spinal implants, titanium has the greatest capacity for osseointegration. Osseointegration results in a smoother, more even distribution of the load between the implant and bone.11 As a general rule, the biocompatibility of metallic materials is closely related to corrosion resistance.
The surface characteristics of an implant material may affect its material properties. For example, the fatigue resistance of a metal may be enhanced by the process of shot peening.12 Shot peening is a surface treatment in which small hard pellets are shot against the surface of a metal. This results in a compression deformation of the surface, which in turn results in an augmentation of the number of cycles required to cause failure.13
Fatigue is the process of progressive permanent structural change occurring in a material subjected to repetitive alternating stresses. Fatigue resistance depends on many factors besides shot peening. In general, it increases as tensile strength increases. Annealed titanium has slightly less fatigue resistance than cold-worked 316L stainless steel.
Alternation of the surface characteristics of an implant may be used to increase component–component friction and thus enhance resistance to component–component failure (see Chapter 14). An example of this is the use of a knurled surface on the Cotrel-Dubousset rod (Fig. 13.3). The combination of the set screw attachment mechanism and the coarse, rough surface of the knurled rod creates a high-friction component–component interface.

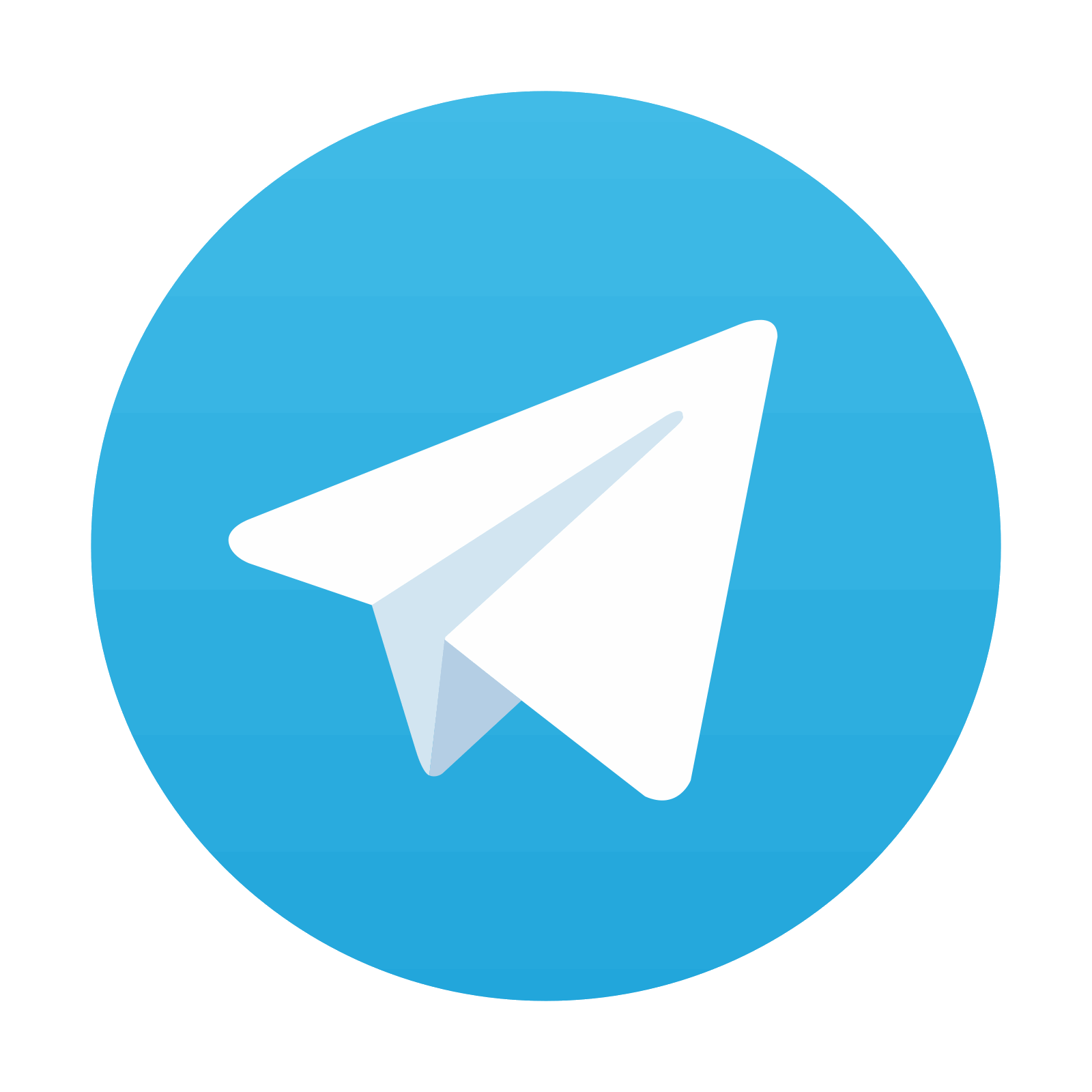
Stay updated, free articles. Join our Telegram channel
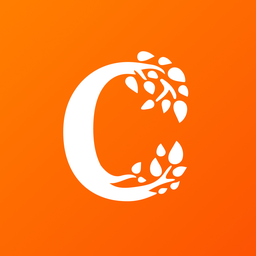
Full access? Get Clinical Tree
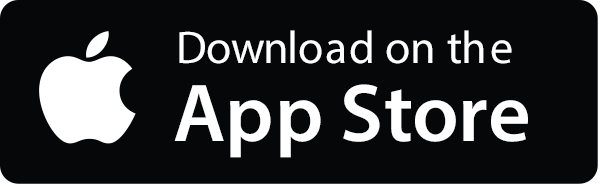
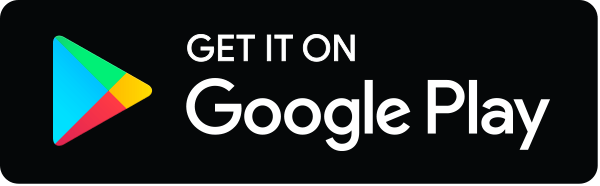
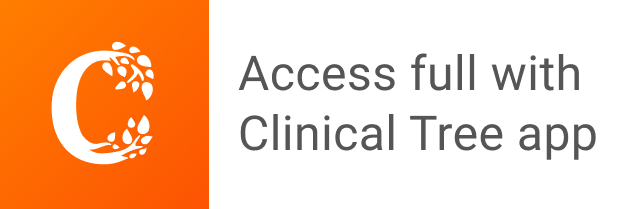