15 Implant–Bone Interfaces
A variety of interfaces between bone and surgically applied implants are used clinically. In spine surgery, there are five fundamental types of implant–bone interfaces: (1) abutting (e.g., interbody bone, interbody acrylic, cages); (2) penetrating (e.g., nail, staple, screw); (3) gripping (e.g., hook, wire); (4) conforming (e.g., acrylic); and (5) osseointegration (e.g. titanium, ceramics).
Within these categories are subcategories and implant variations. The biomechanical principles involved range from the very simple, such as those for abutting interbody implants, to the very complex nuances of screw–bone interfaces. Each category is considered separately, with accompanying theoretical and biomechanical information.
15.1 Abutting Implant–Bone Interfaces
The most common location for the placement of abutting implants is the interbody region. Their application elsewhere, on or within the vertebra, makes little sense. For an abutting construct to be effective, it must bear a load. Because the interbody region is the approximate region of the neutral axis (see Chapter 12), and because most of the axial load is borne in this region, an interbody location is the most appropriate for the placement of abutting implants.
Abutting implants, by their nature, distribute loads over a relatively large surface area of contact. A surgeon would not usually select a slender interbody implant because it would likely knife its way through the relatively soft cancellous bone of the vertebral body. The placement of an interbody implant in close approximation to the end plate (where bone is more compact and thus more able to resist compression) may be desirable (see Chapter 6). Such a strategy takes advantage of the boundary effect.
Specific information on the biomechanics of such implants is lacking. All other factors being constant, however, the larger the surface area of contact between the implant and the bone, the more effective the implant’s resistance to axial loads. The axial load-resisting capacity is, in theory, directly proportional to the surface area of contact. The larger the surface area of contact with the end plate of the interbody–abutting implant—be it bone, acrylic, or a metal—the more effective it will be in achieving one of its most important goals: to resist applied axial loads (Fig. 15.1).

Another goal with an abutting interbody implant is for the implant to remain in the desired interbody location; thus, load bearing is optimized, the chance of neural impingement is minimized, and the chance of subsequent spinal deformation is also minimized. This often necessitates the use of an adjunctive implant component. For example, interbody acrylic implants may be applied with a rigid wire stabilizer that penetrates the end plates of the adjacent and supported vertebral bodies (Fig. 15.2a). This minimizes the chance of implant migration. Some fixators employ spikes at the terminal bone-contacting surfaces in order to achieve the same result (Fig. 15.2b). Bone graft struts are often positioned in a deep mortise or fashioned in a conical shape at the termini. These maneuvers also minimize the chance of implant (interbody bone strut) migration.

There are two types of cage interfaces with the vertebral body end plate: (1) flat-faced (Fig. 15.2c) and (2) round-faced (Fig. 15.2d). The former presents a relatively large surface area of contact to the end plate. This effectively prevents pistoning of the implant into the vertebral body. Round-faced cages (e.g., threaded interbody fusion cages) present a round surface to the end plate. Because the end plate is only 1 to 2 mm thick, and because the round-faced cage penetrates the end plate somewhat, the rounded surface of the cage “sees” only a small portion of the end plate (see Chapter 23 and Fig. 15.2d).
Significant regional end plate strength and stiffness characteristics exist. The center of the end plate, where interbody implants are commonly positioned, is the weakest portion of the end plate. The wall (cortex) of the vertebral body buttresses interbody implants much better than the center of the vertebral body,1 just as the edge of a tin can is better able to bear loads than the center of the can (Fig. 15.2e, f). One can take advantage of this concept clinically via the use of a fin that abuts the end plate in the region of the cortical margin (see Chapter 23 and Fig. 15.2g).
15.2 Penetrating Implant–Bone Interfaces
Penetrating implant–bone interfaces are of two fundamental types: (1) those without attributes of pullout resistance and (2) those with attributes of pullout resistance. The former type includes nails, spikes, and staples. The penetrating adjuncts of abutting implant–bone interface implants are examples of this type. The latter type includes screws and penetrating implants that change configuration on placement into bone (e.g., expanding tip screws).
15.2.1 Penetrating Implant–Bone Interfaces without Pullout Resistance
Nails, spikes, and staples are seldom used as sole methods of implant–bone interface in clinical practice. This is partly because of their relative inability to resist dislodgement; their capabilities for pullout resistance are nearly nil. They usually function as adjuncts (stabilizers) for implants (e.g., as adjuncts for interbody axial load–bearing implants), either as the cantilever components of rigid, constrained implant systems for axial load bearing (fixed moment arm cantilever beam; see Chapter 17) or as the cantilever components of terminal three-point bending constructs (posts; see Chapter 17 and Fig. 15.3).

15.2.2 Penetrating Implant–Bone Interfaces with Pullout Resistance: Implants That Change Configuration after Insertion
Implants that change configuration after insertion into bone have the capacity to resist pullout (Fig. 15.4). They are not commonly used in clinical practice; therefore, little biomechanical information is available.2–4 The available information, however, suggests that expanding tip screws effectively increase pullout resistance, particularly in osteoporotic bone.2,4,5 Adding a nut on the opposite side of a bicortical vertebral body screw significantly increases pullout resistance.6,7

15.2.3 Penetrating Implant–Bone Interfaces with Pullout-Resistant Screws
Most of the information available on implant–bone interfaces addresses screws. This parallels the frequency of their clinical use. In fact, screws, either alone or as components of more complex spinal implants, are being used clinically with increasing frequency and in increasingly broad applications. A relatively thorough knowledge of screw anatomy, screw interactions with bone, and screw biomechanics is mandatory for the effective and safe use of screws.8,9
Screw Anatomy
A screw has four basic components: (1) the head, (2) the core, (3) the thread, and (4) the tip (Fig. 15.5). Each component can be altered to achieve a specific desired clinical effect.

The Head
The head of the screw resists the translational force created by rotation of the thread through the bone at the termination of screw tightening (Fig. 15.6). The screw head, therefore, should be designed to abut the underlying surface optimally. If this surface is medullary cancellous bone, a wide head is necessary to minimize the chance of pull-through. A smaller diameter is required for cortical bone. If the underlying surface is metal, as with a dynamic or semiconstrained screw–plate system, the undersurface of the screw head should conform to the trough in the plate (i.e., it should have a rounded undersurface). This usually permits toggling. On the other hand, if toggling is not desired, a flat undersurface that abuts the flat surface of the plate may be desirable. Obviously, because of the significant deformation resistance of metal compared with bone, the diameter of the head can be smaller with metal-on-metal applications than with metal-on-bone applications (Fig. 15.7).


Once the screw head is brought into contact with the underlying surface during tightening, either or both of two sequelae will result from further tightening of the screw: (1) screw thread–bone interface failure (stripping, or pullout) and (2) deformation of the underlying surface against the undersurface of the screw head.
The Core
The core (inner diameter) provides resistance to fracture in the form of resistance to cantilever bending loading that is perpendicular to the long axis of the screw and torsion. In clinical practice, the torsional strength of the screw is relatively insignificant. However, screws frequently must bear substantial cantilevered loads (loads oriented perpendicular to the long axis of the screw; Fig. 15.8). Hence, bending strength is of considerable importance. Bending strength is proportional to the section modulus (Z) and is defined by the following equation (see Chapter 2):
Equation s. Kap.
in which D = core diameter. Therefore, screw (or rod) strength is proportional to the cube of the core diameter. As the core diameter increases, the strength of the screw increases exponentially. This is especially significant for the core diameters commonly used clinically (Table 15.1).10 Note that the difference in strength between a screw with a 5.0-mm core diameter and one with a 6.0-mm core diameter screw is nearly twofold (125 vs 216). Therefore, the largest screw diameter allowed by the local bony anatomy should be used, so that the likelihood of screw failure (fracture) can be minimized.11 This principle is difficult to apply when the pedicles are narrow, as is usually the case in the thoracolumbar region, which underscores, in part, the biomechanical and clinical problems associated with pedicle fixation in this region. In view of the simplicity of the mathematical relationship between screw diameter and screw strength, it is not surprising that most implant systems have similar attributes.12

Core Diameter (mm) | Relative Strength (cube of core diameter) |
3.0 | 27.0 |
3.5 | 42.9 |
4.0 | 64.0 |
4.5 | 91.1 |
5.0 | 125.0 |
5.5 | 166.4 |
6.0 | 216.0 |
6.5 | 274.6 |
7.0 | 343.0 |
7.5 | 421.9 |
8.0 | 512.0 |
8.5 | 614.1 |
9.0 | 729.0 |
Stress reduction osteoporosis results from stress shielding associated with the use of very rigid implant systems (see Chapter 19 and 29). It is intuitive that shielding bone from applied loads may result in demineralization. This indeed occurs, but the stiffness and stability imparted to the spine by the implant more than compensate for this phenomenon.13 With less rigid systems, movement at the screw–bone interface may occur. Movement at the screw–bone interface causes the screw to become enveloped with fibrous tissue.14 This parallels the degradation of the screw–bone interface.
The Thread and Tip
Strength is proportional to screw core diameter (minor diameter). Outside (outer) diameter (major diameter), on the other hand, is more important as a determinant of screw pullout resistance. The depth of the thread may be even more important in this regard (Fig. 15.9).

Three types of screws are used in spinal surgery: machine screws (cortical screws), self-tapping machine screws, and wood screws (cancellous screws). Cortical screws are used in hard, relatively incompressible bone. Their shallow threads minimize bone compression during screw insertion. The problem of pathologic bone compression by the screw during insertion is eased by pretapping the hole for the screw. For a cortical bone screw to have maximal pullout resistance, pretapping is optimal. Tapping carves threads into the wall of the bone. The cutting edges of the tap screw perform this task.
Two characteristics of a tap screw are fundamental to its success: a tapered tip and a full-length flute. The tapered tip helps to align the screw in the desired direction by directing it down into the predrilled hole. The full-length flute gathers bony debris carved from the wall of the drill hole by the tap screw (Fig. 15.10a). This is facilitated by periodically loosening the screw by approximately one-quarter to one-half of a turn during tightening, which allows the bony debris to collect in the flute. Tapping has been shown to decrease pullout resistance in osteoporotic bone. This phenomenon is less relevant in bone that is not osteoporotic.15–17

Self-tapping screws obviate the need for this multistep process. A leading-edge flute is built into the tip, allowing debris to accumulate within its confines. The shorter flute of self-tapping screws cannot accommodate all the debris created (Fig. 15.10b). Thus, the drill holes should be larger with self-tapping screws (slightly larger than the core diameter of the screw) to facilitate the accumulation of debris around the threads.
Pretapped non–self-tapping and self-tapping screws, if used properly, provide similar pullout strengths. Furthermore, the pullout strength of both pretapped non–self-tapping screws and self-tapping screws is not significantly affected by multiple insertions and removals in cortical bone.18
Cancellous (wood) screws are used in softer material—that is, in cancellous bone. The compression of cancellous bone by the screw during insertion increases the density of the bone that surrounds the screw, and thus its pullout resistance. In cortical bone, compression during screw insertion causes microfractures that decrease bone integrity. Although pretapping is desirable in cortical bone, it is less desirable in cancellous bone. In fact, in cancellous bone, tapping weakens the implant–bone interface.
Pedicle screws rarely obtain cortical purchase within the pedicle.19 Because tapping weakens the implant–bone interface in cancellous bone, the tapping of pedicle screw holes is of questionable value. However, in cortical bone, bone microcracking around screw threads is greater with untapped than with tapped screws.20 Therefore, in cortical bone, untapped screws loosen more frequently than tapped screws. Pretapping is thus desirable in cortical bone.
Pullout Resistance
Pullout resistance correlates with insertional torque.6,15,17,21,22 This has been defined by the following equation:
Equation s. Kap.
where y = insertion torque in newton-meters and x = pullout resistance in newtons. Insertional torque is increased by employing conical-inner-diameter screws. The use of conical-inner-diameter screws renders the aforementioned equation useless. Insertional torque under such circumstances potentially gives one a false sense of security associated with the false notion that pullout resistance correlates with insertional torque. As mentioned above, the main determinants of screw pullout resistance are the major diameter of the screw23 and thread depth. Other important factors are extent of cortical purchase, depth of screw penetration, and thread design. The several threads nearest the head of the screw bear most of the load transferred from bone during pullout stressing. Therefore, proximal cortical “purchase” is very important regarding pullout resistance. Of secondary importance is the depth of penetration of the screw within the bone.24 Third, distal cortical purchase seems to be even less important in this regard.25,26 This last point is understandable in view of the fact that the greatest load is transferred by the most superficial threads.
Thread design also plays a role in screw pullout resistance. Two factors dominate this aspect of screw mechanics: thread pitch and thread shape. Thread pitch is the distance from any point on a screw thread to the corresponding point on the next thread. This is equal to the distance a screw advances axially in one turn (lead). A fundamental rule of thumb of screw biomechanics is that pullout resistance is proportional to the volume of bone between the threads. This, however, is a significant generality (see the following). As previously mentioned, increasing the thread depth increases pullout resistance. Thread depth obviously correlates with bone volume between threads. Similarly, the pitch of the thread is proportional to the volume of bone between threads and thus to pullout resistance.
Altering the shape of the thread can increase or decrease the interthread volume. For example, flattening or reversing the angle of the following edge of the thread further increases interthread volume (by decreasing metal volume) and results in an even greater increase in pullout resistance. Screw toe-in (triangulation) also contributes to pullout resistance if the two sides of the construct are rigidly affixed to each other by a cross member (Fig. 15.11).27

The factors that determine screw pullout resistance are obviously complex.23,28,29 Chapman and colleagues30 have attempted to quantify these factors objectively via the following equation:
Equation s. Kap.
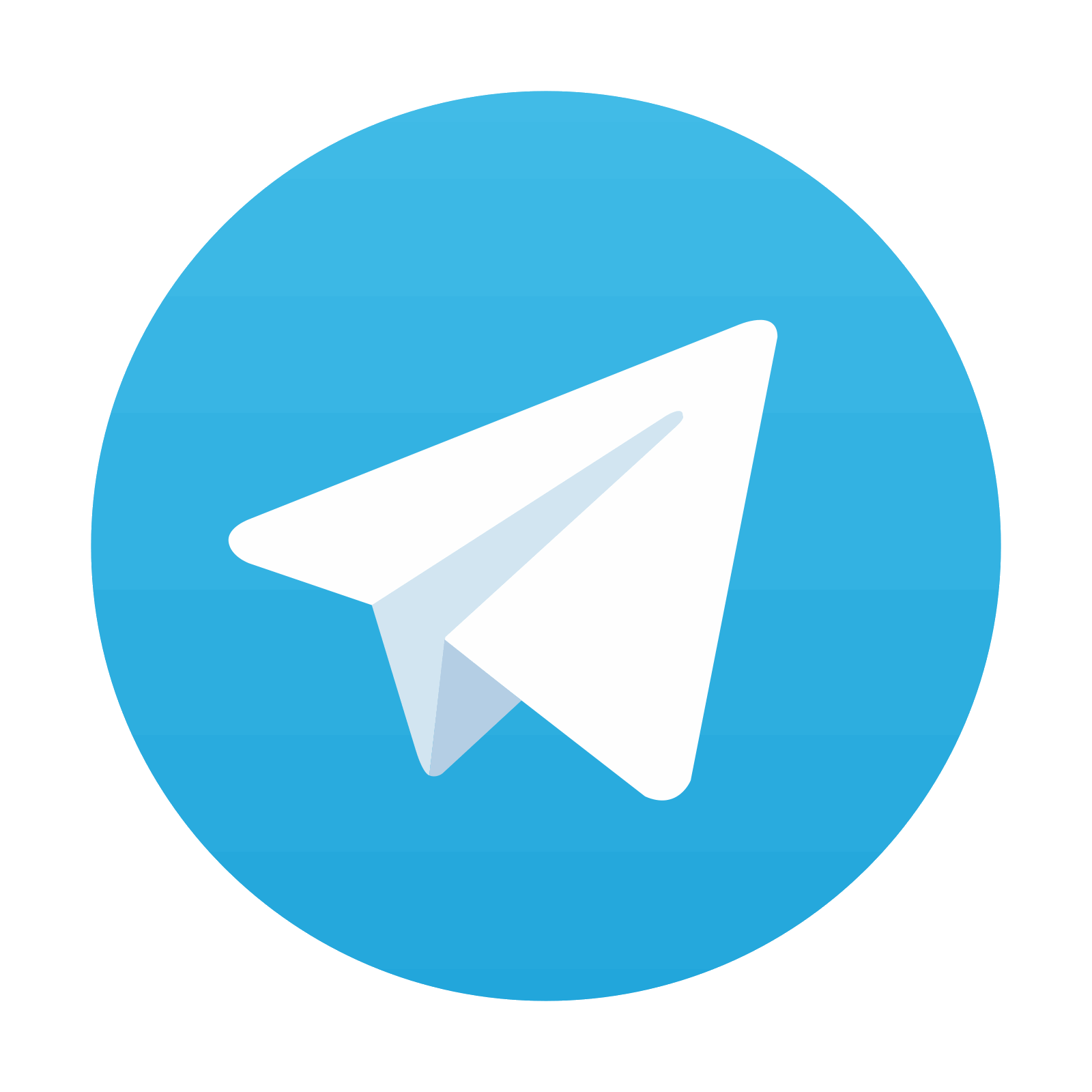
Stay updated, free articles. Join our Telegram channel
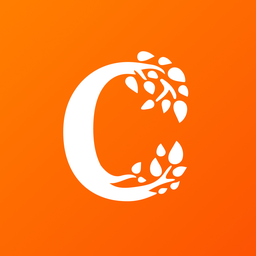
Full access? Get Clinical Tree
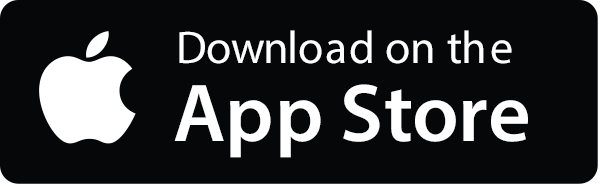
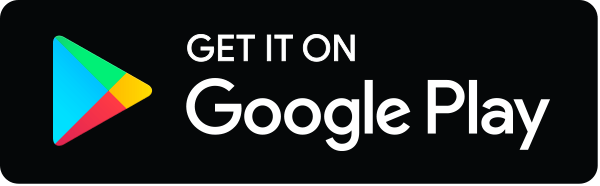
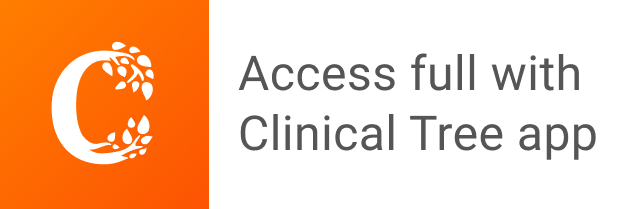