16 Laser-Induced Thermal Therapy for Medically Refractory Epilepsy
Abstract
Minimally invasive approaches have become a popular part of medicine. Neurosurgery has seen a massive influx of new technologies focused on achieving improved outcomes through smaller and smaller incisions. Epilepsy can be a complex and challenging disorder to treat, and surgical interventions are often viewed as a last resort. In such cases, the goal is identification of the epileptogenic source and safe removal of that source. For the past 50 years, surgical approaches have involved open approaches often requiring retraction or removal of key structures. Recently, the development of minimally invasive ablative techniques has been applied to neurosurgery, particularly magnetic resonance–guided laser-induced thermal therapy (MRgLITT). MRgLITT has been applied to focal epileptogenic disorders such as mesial temporal lobe epilepsy, focal dysplasia, and hamartomatous disease. In this chapter, we explore the history, physics, surgical steps, and to-date outcomes for MRgLITT in the treatment of medically refractory epilepsy.
16.1 Introduction
The challenges associated with management of epilepsy are known to all clinicians; often over 30% of medically treated patients fail conventional therapy. 1 , 2 These cases of drug-resistant epilepsy (DRE) often leave patients with significant disability affecting them medically, psychosocially, and financially. Focal epileptic disorders such as mesial temporal lobe epilepsy (MTLE), focal dysplasia, and hamartomas are often notorious for being refractory to medical treatment. In such cases, surgical interventions can often achieve seizure freedom. Although open approaches such as lesional resection, anterior temporal lobectomy (ATL), or more selective approaches such as selective amygdalohippocampectomy (SAH) have been the standard, minimally invasive options are being explored. Radiofrequency ablation and, more recently, laser ablation have shown promising results in the treatment of these challenging cases. 1 , 2
Magnetic resonance–guided laser-induced thermal therapy (MRgLITT) has experienced a surge in utilization in the past decade particularly for neurosurgical applications. 1 , 2 , 3 , 4 As a minimally invasive option that can result in meaningful outcomes, MRgLITT has become a potentially valuable tool in the neurosurgical armamentarium. Intracranial targets such as brain tumors, pain generating circuits, and epileptogenic foci have been targeted. 4 , 5 The application of MRgLITT to focal seizure disorders has been of particular interest. Surgical approaches to focal epilepsy have involved a wide range of techniques in the past. From focal resection to large-scale hemispherectomy, epilepsy surgery often involves complex planning and requires a high degree of surgical skill in order to prevent unintended damage. The minimally invasive approach offered by MRgLITT provides an alternative to open surgery in select cases as it involves a small burr hole and trends toward shorter hospital stays. 3 , 4 MRgLITT works by thermal ablation, essentially melting tissue in situ, and the procedure is largely performed in the magnetic resonance imaging (MRI) suite. The purpose of this chapter is to provide an overview of the history, surgical steps, mechanisms, and current insights into MRgLITT-based management of epileptic disorders.
16.2 History
Surgery for epilepsy has been utilized since the early 20th century when Penfield incorporated electroencephalography (EEG) into the operative procedure. 6 , 7 His initial work focused on anterior temporal lobe resection with EEG guiding additional resection. He met limited success and then through further study, fine-tuned his approach. Penfield then included resection of mesial temporal lobe structures and his results were far more successful. Penfield emphasized the critical point that along with temporal lobectomy, resection of mesial temporal structures including the amygdala and hippocampus was important. This led to multiple other approaches such as transsylvian and transcortical techniques. 7 However, all of these open approaches carried risk of speech, neuropsychological, and cognitive impairment. Furthermore, open approaches involve a larger craniotomy and relatively longer hospital stay. Over several decades, the development of various selective approaches has had the goal of achieving seizure freedom while minimizing collateral damage. The development of MRgLITT has been occurring with this same goal in mind.
Minimally invasive approaches to ablate intracranial lesions have existed for several decades, and the use of lasers to achieve thermal ablation was first described by Bown in 1983. 8 He first proposed laser ablation with the notion that laser energy could be transmitted via flexible optic fibers to target any lesion in the body without damaging surrounding healthy tissue. The advantages of laser ablation in neurosurgery became immediately apparent. However, the widespread application of laser ablation was hindered by the inability to monitor thermal energy deposition and the ablated region intraoperatively. In 1988, Jolesz et al suggested the use of MRI to map the spatial and temporal effects of Nd:YAG laser on tissues. 9 Their group suggested that the high sensitivity of MR to changes in mobility and distribution of tissue water makes it particularly suitable for monitoring thermal energy deposition. Later in 1994, Kahn et al reported that intraoperative MRI is feasible and effective in monitoring treatment response of laser ablation. 10 However, it was not until later in the 1990s when MRI thermography was developed to allow real-time monitoring of temperature changes. 11 This advancement allowed for commercial development of MRgLITT systems and the two modern MRgLITT systems came into wide use. The Medtronic (Visualase; Medtronic Inc., Minneapolis, MN) and Monteris (Neuroblate; Monteris Inc., Minneapolis, MN) systems are the two systems in existence today. Each carries the same principle: laser energy delivered through a diode fiber with real-time MRI monitoring of thermal damage. The Medtronic (Visualase) system offers potential advantages in wavelength of energy as the 980 nm wavelength has been shown to be associated with faster and with larger ablation zones. 7 The Monteris system offers advantages in terms of directional laser firing, allowing finer control of therapy delivery. Regardless, both demonstrate significant historical strides from the initial work by Bown. 8
16.3 Relevant Pathophysiology
The structures involved in MTLE include the amygdalohippocampal complex and associated pathological changes (see ▶Fig. 16.1). Histologically, there are changes in the cytoarchitecture and composition of the hippocampus such as sclerosis and degeneration. 12 MTLE tends to follow insults early on such as febrile seizures, infections, and trauma. The exact pathophysiological mechanisms of epileptogenesis are not entirely understood; however, a prevailing hypothesis suggests adenosine fluxes may play a role. Insulted glial cells may experience increased levels of adenosine and subsequent upregulation of adenosine kinase. 12 After a certain threshold is reached, irregular synaptic activity occurs. Another hypothesis involves inflammatory mediators such as cytokines that trigger glial cell damage. 12 However, no single hypothesis has been proven.

Other forms of focal epilepsy, such as those due to focal cortical dysplasia and hamartomatous lesions, have different mechanisms than those postulated for MTLE. For focal cortical dysplasia, it is thought that the abnormal neurons have increased expression of NMDA receptors and a corresponding enhanced excitability. 13 In addition to receptor changes, there is thought to be disorganized synaptic connectivity in these regions, therefore favoring epileptogensis. 13 Intracranial recordings have suggested that the epileptogenic zone may extend beyond the identifiable lesion boundaries. This extension complicates surgical management, especially in the context of MRgLITT. 13 The seizures seen in hypothalamic hamartomas are described as gelastic (laughing) seizures. The pathogenesis of seizures in hamartomatous cases is thought to occur through multiple processes such as abnormalities in hormonal expression, local irritation of normal tissue, and may originate in the abnormal tissue itself. 14
16.4 Physics and Hardware Considerations
Lasers in medicine are often used for treatment and frequently as surgical devices. From a purely physics perspective, they represent nonionizing radiation delivered through a single beam of energy. A fine balance between absorption and penetration is critical in achieving efficient heating of tissue. 4 , 11 As brain tissue is a water-dominant medium, light wavelengths that penetrate through such a medium are key. But it is important to note that brain tissue is not entirely water; tissue perfusion, protein content, and proximity to fluid bodies all affect laser absorption and penetration. Wavelengths of light near the infrared spectrum are better in terms of photon scatter over absorption, leading to more rapid heating. 4 , 11
Contemporary surgical lasers used for brain interventions are highly mobile with a small size and are classified as class IV solid-state diode lasers. Output ranges in the 2- to 40-W range, typically in the 10- to 20-W range for most ablation procedures. 4 , 11 Oxyhemoglobin and deoxyhemoglobin absorb optimally at the lower range of infrared spectrum (850–1,100 nm), while water is better at the higher end. 4 , 11 This is the essential reasoning behind the choice of a 980-nm (Visualase) and 1,064-nm (Monteris) wavelengths in modern MRgLITT systems. Beyond this range, absorption drops and there is a reduced rate of tissue heating for similarly delivered power. 4 , 11
Laser energy delivery is accomplished via long and flexible optical fibers, with cladding materials, on the order of 400 to 400 µm in diameter. 4 , 11 These fibers extend beyond 10 m as they must traverse from the MRI control room into the MRI scanner bore to the patient; this is the typical setup for modern MRgLITT systems. As high temperatures can damage the laser fibers, modern systems utilize built in cooling mechanisms. Typically these mechanisms consist of a coaxial cooling system using either CO2 gas or flowing water (saline). With the cooling system in place, overall diameter of the fiber system ranges 1.8 to 3.3 mm, depending on the type of laser used.
The MRI guidance is a key feature of MRgLITT and therefore accurate representation of thermal damage is critical to this procedure. The monitoring of temperature is accomplished via proton resonance frequency (PFR) shift. 4 , 11 The MRgLITT software of modern systems can capture this PFR shift on gradient recalled echo (GRE) images and convert it into temperature. The sensitivity of this system is approximately 0.01 ppm/°C and the resultant data can be overlain on serially acquired MR images. Goal temperature is kept in the less than 100°C range at the interface of the catheter and target; at the periphery, where normal tissue meets target tissue, temperature is kept in the less than 60°C range to prevent irreversible damage. The temporal acquisition of temperature data can be translated into a damage estimate using models such as the Arrhenius equation. 4 , 11 The PFR shift predicted temperature and time of exposure are the variable inputs into this model.
16.5 Surgical Steps
Planning based on the preoperative MRI is crucial for optimal targeting and ablation. Once the entry point, target, and trajectory are identified, a burr hole is created; next, the catheter is inserted followed by laser ablation. Although more than one MRgLITT system exists, the general steps are identified below (▶Fig. 16.2, ▶Fig. 16.3). Although the specific steps have been published by Patel et al previously, they were generalized to all intracranial cases of MRgLITT using the Medtronic Visualase system. 4 When using MRgLITT for epilepsy, planning is highly dependent on the type of epileptic source (such as MTLE, focal dysplasia, hamartoma). For MTLE, the laser is often placed from an occipital burr hole with the laser tip extending to the amygdalohippocampal complex. A series of sequential “pull-backs” are performed to create a column of ablation. For focal dysplasia, and other lesions, the entry and trajectory are entirely dependent on anatomic location. In cases of hypothalamic hamartomas, an entry in the frontal bone is chosen, similar to that for a deep brain stimulation lead. The general steps are described below:


Registration and burr hole creation—An entry and target point are identified and this guides trajectory planning. Registration may be performed as trajectory-guided platform, frameless, and stereotactic frame based. Additionally, it may also be performed with robotic assistance. Surface landmarks, gadolinium fiducials, or implanted fiducials may be used in frameless cases. In certain instances, trajectory guide platforms may be used; these are fixed to the skull and used as a stereotactic reference, as seen with the ClearPoint system (MRI Innovations, Irvine, CA). Typical stereotactic frames may also be used for MRgLITT with planning similar to that for a stereotactic biopsy. After the trajectory is planned, a burr hole is made at the intended entry site. A small bone anchor holds the laser catheter in place.
Laser catheter placement—Once the skull and dural openings are complete, and the bone anchor is in place, the laser catheter is marked to the measured trajectory length. Of note, it is important to measure the trajectory length starting from catheter entry point on the anchoring device/bolt and any additional guiding cannulas. Once the laser catheter is at target, the anchor is locked.
Laser ablation—The laser and its cooling line is connected to the MRgLITT workstation in the MRI control room. Once a series of MR images confirms catheter placement, the optimal treatment plane is chosen. This treatment plane will be the reference image for the procedure. Secondary and tertiary planes may also be chosen (depending on the MRgLITT system), optimally in orthogonal orientations. Once a test dose confirms appropriate heat delivery, the procedure is performed. A temperature map and damage estimate map may be overlain on the reference MR image to determine extent of ablation (see ▶Fig. 16.4).
Postprocedure—Once ablation is satisfactory, the patient undergoes an immediate post-MRgLITT formal MRI and then is removed from the MRI machine. The laser catheter, cooling catheter, and bone anchor are removed. A single absorbable suture is usually used to close the incision. Postoperatively, an MRI is obtained at 24 hours. Patients are often discharged within 24 to 48 hours.

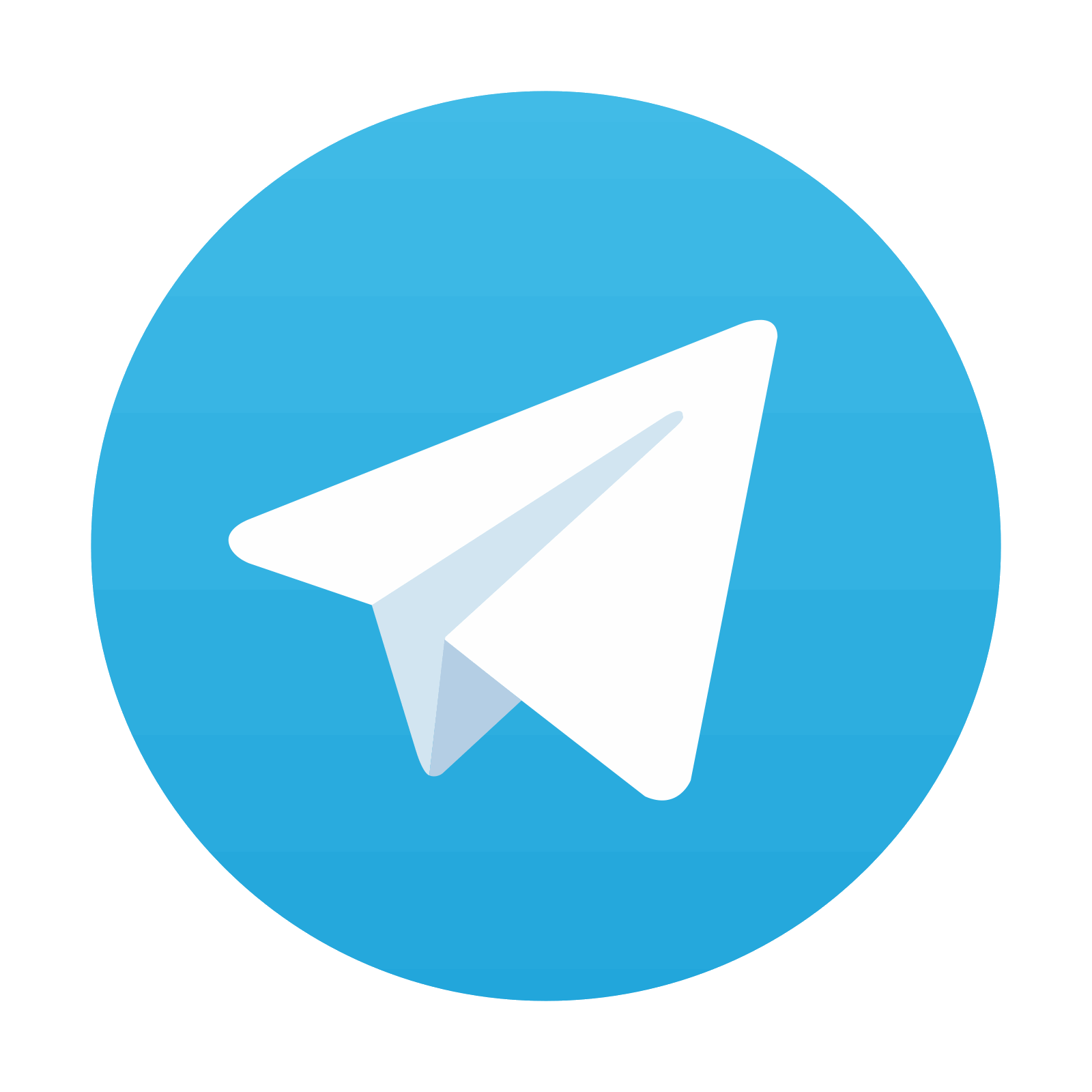
Stay updated, free articles. Join our Telegram channel
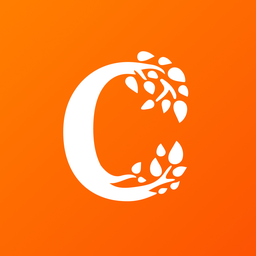
Full access? Get Clinical Tree
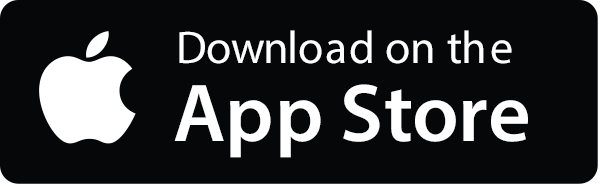
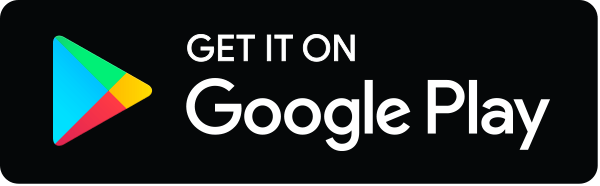