16 Qualitative Attributes of Spinal Implants: A Historical Perspective
This chapter, for the most part, is a true carryover from the second edition of this book. It is re-presented, predominantly from a historical perspective. Many of the techniques presented in this chapter are not employed today. Yet, lessons learned from times gone by can be effectively applied today. Many of the lessons learned via the use of the techniques presented in this chapter set the stage for the development and effective utilization of modern techniques.
Spinal implants are either predominantly rigid (constrained) or predominantly dynamic (semiconstrained), and they impart distractive, compressive, or neutral axial forces to the spine (Table 16.1), as well as forces around or perpendicular to the long axis of the spine. Rigid implants are used to achieve rigid fixation of the spine. Dynamic implants allow some intersegmental movement, which eases (offloads) stresses placed elsewhere in the system (usually at the implant–bone interface) and also increases bone healing–enhancing stresses (à la Wolff). These factors are the determinants of the mode of application.
Most spinal implants apply forces to the spine in a complex manner. The complex nature of force application can be simplified by considering the six mechanisms of implant-derived force application discussed in Chapter 17: simple distraction, three-point bending, tension-band fixation, fixed moment arm cantilever beam fixation, nonfixed moment arm cantilever beam fixation, and applied moment arm cantilever beam fixation.
Rigid | Dynamic | ||
Dorsal | Ventral | Dorsal | Ventral |
Distraction | Distraction | ||
Neutral | Neutral | Neutral | Neutral |
Compression | Compression | Compression | Compression |
It is important to recognize that there is no truly neutral spinal implant. For example, if an implant is placed in a neutral mode at the time of surgery, its characteristics soon change when the spine is loaded (e.g., via assumption of an upright posture after surgery; Fig. 16.1a, b). Stated differently, implants are loaded differently under differing loading conditions. Hence, the mechanism by which they apply and resist loads differs according to the loading conditions. This is exemplified by considering a ventral cervical plate associated with an anterior cervical discectomy, fusion, and plating. In the supine position, the plate may not be loaded. In the upright position, it functions as a fixed moment arm cantilever beam fixator and as a distraction device when axially loaded. When the spine is extended, it resists such by functioning as a compression device (tension-band fixator). Hence, it functions very differently under differing loading conditions. The failure to recognize this phenomenon is perhaps the most common cause of surgeon-generated (iatrogenic) construct failure (surgeon failure; Fig. 16.1 c–e).

Thus, many spinal implants that are initially placed in a neutral mode eventually bear axial loads (i.e., they function as a distraction device when axial loads are applied). In addition, they can function as tension-band fixators when they are loaded via spinal extension. For the purpose of consistency, and with this in mind, neutral devices are considered herein as those placed in a neutral mode at the time of surgery (i.e., without distraction, compression, three-point bending, or cantilevered force application).
The “desired axial forces applied” by the spine surgeon using spinal implants are discussed herein. The “axial components” of these desired forces can be broken down into 10 clinically used categories. They include both dorsal and ventral techniques (five varieties of each) for spinal instrumentation. The dorsal categories are the following: (1) rigid distraction (with or without three-point bending); (2) rigid neutral; (3) rigid compression (including most tension-band fixation constructs); (4) dynamic neutral fixation (including most cantilever bending constructs with nonfixed moment arms); and (5) dynamic compression (including some tension-band fixation constructs—that is, springs). The ventral categories are the following: (1) rigid distraction (simple distraction or interbody buttressing); (2) rigid neutral (cantilever bending constructs with fixed and nonfixed moment arms); (3) rigid compression; (4) dynamic neutral fixation (placement of an interbody strut without distraction); and (5) dynamic compression (see Table 16.1). There are no true dynamic distraction devices (ventral or dorsal) readily available for clinical use.
A “clean” separation of these desired axial force applications into their respective categories is often impossible. The attempt to categorize them here, therefore, is somewhat artificial and is presented to facilitate an understanding of spinal implants and to foster the matching of what the surgeon expects from the implant (desired force application) to what is actually achieved (achieved force application).
Newton’s third law of motion states that interactions between objects result in no net change in momentum; in other words, for every action, there is an equal (in magnitude) but opposite (in direction) reaction (see Chapter 2). Spinal instrumentation constructs, as well as all other methods of force application in nature, obey this law. Because spinal instrumentation constructs do not “move” the spine after insertion, it may be presumed that all forces applied to the spine are applied in pairs—that is, because spinal movement does not occur, all forces must be balanced, with a net force of zero (see Chapter 2). Therefore, two equal (but opposite in direction) linear or moment-creating forces act on the instantaneous axis of rotation (IAR) of a vertebral spinal segment when a spinal implant either applies a force to the spine or resists spinal movement (Fig. 16.2). The importance of this concept cannot be overstated. It is considered, with the variety of desired force applications (modes of application), in the pages that follow.

Herein, specific attention is paid to axial force application (compression or distraction). Flexion–extension, lateral bending, and translational force applications are more complex and less frequently theoretically considered than compression and distraction forces. In the spirit of simplicity, axial force applications are emphasized in this chapter. Flexion–extension, lateral bending, and translational force application, however, are discussed where appropriate, both here and in the chapters that follow.
16.1 Rigid Fixation
The goal of rigid spinal instrumentation is absolute immobilization of the spine. Under most circumstances, this goal (desired force application) cannot be completely achieved. Because bone is a biological material, it deforms and reforms according to the stresses placed on it. Therefore, even the most rigid of devices allows some movement. The range of this movement often increases with time, as the implant–bone interface becomes looser. If such movement occurs with enough repetitions and with enough force, it will eventually cause failure at the implant–bone interface, unless at least one of two conditions exists: (1) bony fusion occurs (taking over the weight- and stress-bearing burden from the construct–bone interface) or (2) the instrumentation device itself fails (see Chapter 12). The surgeon’s awareness of the “race” between the acquisition of a solid bony fusion and eventual instrumentation failure is critical to the clinical decision-making process (see Chapter 12).
When one recognizes that even the most rigid of implants eventually allows some spinal movement, the distinctions between the various modes of application of spinal instrumentation become poorly defined. Rigid implants, in a sense, eventually become dynamic because of the impossibility of permanent rigid fixation of the bones of the spine.
Rigid fixation does not optimize bony fusion acquisition because of the phenomenon of stress shielding; but if rigid fixation holds rigidly for a sufficient time, bony fusion is usually eventually achieved. Unless the fixation devices are removed, however, the ultimately desired fusion strength may not be realized because of stress shielding and stress reduction osteoporosis. Fusion rates, however, have apparently not been adversely affected by the application of rigid instrumentation constructs. 1 These phenomena, therefore, are more theoretical than real.
16.1.1 Rigid Axial Force Applications
Dorsal Rigid Distraction Fixation
Harrington distraction rod fixation, in years gone by, was the “gold standard” for thoracic and lumbar stabilization for more than 20 years. 2 , 3 Its durability as a favored technique is a testament to its utility. It uses rigid distraction force application, usually combined with three-point bending forces. It provides an opportunity to reduce kyphosis or retropulsed bone and/or disc fragments. This can be accomplished with or without the use of adjuncts to enhance the kyphosis reduction, such as dorsally positioned sleeves. 4 The latter technique uses distraction and a three-point bending mechanism of load application to achieve its effect (see Chapter 15 and Fig. 16.3 a). This is a good example of the complex nature of force application by an implant. A spacer, such as a sleeve, can be used to exaggerate the ventrally directed force at the fulcrum (three-point bending; Fig. 16.3b). This increases lordosis. Other strategies can be used to maintain a lordotic posture. These include cross fixation and the use of intermediate points of fixation (multisegmental fixation; Fig. 16.3c). Square-ended attachment sites of the rod to the hook have been used as a modification of the Harrington distraction system. 5 The latter all maintain the lordotic posture by using contoured rods and maintaining their orientation. The sleeves allow a straight rod to be used to accomplish the same goal (see Fig. 16.3b). Today, the use of screw–rod fixation systems obviates the need to consider the aforementioned. Nevertheless, an understanding of the principles involved is critical to the development of a sound foundation of spine surgery related “biomechanical awareness.”

Failure at the hook–bone interface was a common problem associated with Harrington distraction rod application. 6 This is a function of the nature and magnitude of the forces applied at the hook–bone interface.
The Harrington distraction rod may also fail (fracture) at the proximal ratchet of the rod and at the sites of rod contouring because of metal fatigue (stress risers). The surgeon can reduce the risk for metal fracture by not contouring the rods before placement 7 and by placing the hook as proximally as possible on the rod (i.e., by using the longest rod possible), thus using only a few ratchets. The latter strategy reduces the length of the lever arm (moment arm) between the hook and the first ratchet (the point on the Harrington distraction rod construct at which the greatest stress is placed).
Dorsal spinal distraction has several inherent drawbacks (see Chapter 8). First, axial ligamentous resistance is required for the Harrington distraction rod technique to be effective (Fig. 16.4) because a “claw” configuration (which circumferentially “grips” a lamina) is not used with the standard Harrington system (see Chapter 11). If significant ligamentous laxity is present, a counter-resistance does not exist, and the hook may dislodge because of the absence of effective counter-resistance.

Another inherent drawback of dorsal spinal distraction is its inconsistent ability to reduce retropulsed bone and disc fragments, even when applied in combination with a three-point bending force (ligamentotaxis or annulotaxis). This may contribute to the lack of neurologic improvement observed with this technique in comparison with nonoperative approaches. 2 Although an intact anterior longitudinal ligament augments the efficacy of this technique and may be a requirement for the appropriate application of Harrington distraction rod fixation, it may hinder the reduction of retropulsed bone and disc fragments. This technique, therefore, may fail because of one or more of three anatomical and pathologic factors: (1) the relative weakness of the posterior longitudinal ligament, (2) the relative strength of the anterior longitudinal ligament, and (3) the frequent occurrence of posterior longitudinal ligament disruption following trauma (see Chapter 8). The anterior longitudinal ligament is often preserved following trauma. Its location allows it to be the distraction-limiting structure (providing axial ligamentous resistance), minimizing the extent of spinal distraction achieved by instrumentation techniques. This is because the ligament itself often prevents the distraction required to reduce these fragments. In addition, spinal cord distraction (especially over a ventral mass) may cause further neural injury by means of a tethering mechanism. 8 , 9 Finally, Dickson et al have shown, as mentioned previously, that neurologic outcome is no better with dorsal instrumentation techniques without an accompanying ventral neural decompression procedure than it is with postural nonoperative treatment. 2 The end result, “no neurological improvement,” may reflect the averaging of neurologic improvement after neural decompression (associated with the instrumentation technique in some cases) and a worsening of neurologic outcome or lack of achievement of all potential neurologic recovery arising from distracting the neural elements over irreducible retropulsed ventral bony and soft tissues (see Chapter 8).
Elimination of the normal lumbar lordosis, by using the application of a bending moment with distraction techniques, may similarly cause adverse sequelae (Fig. 16.5). 7 These complications may be minimized by the use of a spacer or sleeve (see Fig. 16.3) or by rod contouring combined with a technique to prevent rod rotation, such as the use of square-ended rods, cross fixation, or the use of intermediate points of segmental fixation with sublaminar wires, hooks, or screws.

Multisegmental fixation can be used to gain the advantage of load sharing. Load sharing involves the distribution of an applied load between multiple components of an implant system and/or between the implant itself and intrinsic spinal elements. The augmentation of Harrington distraction rod fixation, for example, with multiple-level sublaminar wire fixation adjuncts substantially increases stability and decreases the failure rate of Harrington distraction rod fixation. 10 – 17 However, it carries with it the risks associated with the placement of sublaminar wires. 11 , 18 Today, multiple points of fixation are achieved with hooks, or more commonly, screws. All techniques, however, achieve the same biomechanical effect.
Jacobs locking hooks (no longer used today) provide an alternative to simple Harrington distraction rod techniques. 19 Security is provided by locking hooks that help prevent failure at the hook–bone interface with a claw configuration, eliminating the need for intrinsic axial ligamentous resistance. This advantage is also observed with modern day hook–rod and screw–rod systems. The claw configuration allows the use of smaller distractive forces (because ligamentous counter resistance is no longer needed). This, in turn, decreases the chance of failure. Smaller distractive forces with locking hooks can be used because distraction in a nonlocking system is the mechanism by which failure is prevented; thus, intrinsic axial ligamentous resistance is optimally exploited. The greater the distractive force (up to a point), the lesser the chance of hook dislodgement. If the distractive forces are applied to excess, hook insertion site failure will occur.
In selected cases, external skeletal fixation can play a role in spinal trauma management. It can be placed in a distraction posture. However, the risk for infection, the requirement for transpedicular placement, and the less-than-optimal fixation obtained detract substantially from the utility of external skeletal fixation.
Universal spinal instrumentation (USI; systems that apply hooks and/or screws in a multisegmental manner, usually with a rod as the longitudinal member) fixation (placed in distraction) can provide multisegmental rigid distraction. 21 – 25 It achieves substantial stability with a minimal chance of implant failure at the hook–bone interface. This is so because for the following reasons: (1) the ability of the surgeon to apply claws (as with the Jacobs system); (2) the option of employing multisegmental fixation; (3) the option of using wire, hooks, or screws and anchors; and (4) the option of using distraction, compression, neutral, or combinations of force applications. No other type or class of spinal instrumentation provides all of these advantages. Multisegmental fixation distributes the construct’s applied force over multiple segmental levels and achieves results superior to those of previously employed techniques (see following sections “Dorsal Rigid Neutral Fixation” and “Dorsal Rigid Compression Fixation”). 23 Because of the substantial stability and minimal chance of instrumentation failure achieved with modern day multisegmental hook–rod and screw–rod fixation techniques, such systems should be considered the “gold standard” for posttraumatic thoracic and lumbar hook–rod spinal instrumentation. Such techniques involve the use of hooks, rods, screws, and a variety of connection mechanisms to apply multisegmental fixation to the spine.
Dorsal Rigid Neutral Fixation
There are several types of dorsal rigid neutral fixation. They differ widely enough in structural characteristics and techniques of application that each subset is discussed independently.
To minimize the chance of failure of rigid fixation devices (either failure at the implant–bone interface or implant fracture), multisegmental fixation has been used. The Luque rod technique is the historical prototype of dorsal rigid neutral rod fixation. 11 , 17 , 24 , 26 , 27 It provides increased stability by distributing the fixation forces over multiple segmental levels (load sharing); this increases the cumulative fixation (resistance to movement). The distribution of forces decreases the stresses applied to the metal–bone interface at each segmental level. Some dorsal rigid neutral rod fixation devices provide for axial growth when applied before growth potential is achieved (Luque rods). This effect is usually considered a positive attribute of this type of fixation. On the other hand, undesirable settling of the spine may occasionally occur as the rods slide in opposite directions past each other. This can be partly rectified by cross fixing the rod on one side to the rod on the other (Fig. 16.6). One must keep in mind, however, that with Luque rod instrumentation and equivalent techniques, the risks associated with the passage and inadvertent manipulation of sublaminar wires are ever present. 11 , 18 , 28

USI fixation (neutrally placed) can be used in a dorsal rigid neutral mode. Transpedicular screw instrumentation techniques can also be employed in a rigid neutral mode. Modifications of these techniques, for application in the upper cervical spine and the suboccipital region, may occasionally be indicated. 29 The risks of transpedicular screw placement in the cervical region are considered prohibitive by most surgeons, whereas lateral mass plating techniques are considered much less risky and are therefore commonly employed.
Rigid low thoracic and lumbar transpedicular plating and related rodding techniques for spinal instability have been used to provide alternatives to the aforementioned rigid fixation approaches. 23 , 30 , 31 These alternative techniques use either a rod or a plate as the longitudinal member. They provide the rigid application of a plate or rod to transpedicular screws. Although these techniques eliminate the well-known risks of sublaminar wiring, they introduce another threat to neural elements and surrounding structures via the inherent difficulty of placing the screws precisely through the pedicle into the vertebral body, particularly in the thoracic region. With good screw fixation, the constructs that provide true rigid fixation may fail by screw fracture. Because the screws are rigidly affixed to the plates or rods, and because the predominant forces applied to these devices are axial (i.e., perpendicular to the axis of the screw), the majority of the stresses are focused along the screw. They are focused at the screw–plate or screw–rod interface if a constant-inner-diameter screw is employed. This can result in failure at this juncture (see Chapters 2 and 19). 32
Pullout of rigid screw fixation at the screw–bone interface is less likely than with dynamic screw fixation techniques because pivoting of the screw at the plate (as occurs with dynamic, semirigid, semiconstrained, or nonfixed moment arm cantilever devices, which are all relatively synonymous) cannot occur. Therefore, the screw pullout characteristics of rigid neutral spine screw fixation techniques are good, but the metal (screw) failure characteristics are poor. If applied improperly, the implant may be excessively loaded. The applicability of these techniques to situations in which intrinsic spinal ventral weight-bearing ability is impaired (e.g., following trauma) is suspect. 32 Under such circumstances, “load sharing” with a ventral strut or with an intact vertebral body may be appropriate (Fig. 16.7). The sharing of loads between spinal components has been studied by finite element modeling approaches. 33 These data corroborate the efficacy of the “load-sharing” concept.

Rigid screw–rod or screw–plate constructs (fixed moment arm cantilevers; see Chapter 17) function as buttresses. A buttress is defined here as an implant that has the ability to rigidly bear axial loads and resist deformity.
Facet wiring and bone grafting techniques use a bone graft that is wired to the facet at each involved spinal level. 34 This technique provides acute stability but may fail because of wire pullout or fracture of the graft. In addition, remodeling of graft bone at its interface with the wire, resulting in loosening, may occur before the acquisition of solid fusion. The technique is most applicable in the cervical region, where stresses placed on the spine are smaller than in the thoracic and lumbar regions. It is inferior to an interspinous wiring technique because of the shorter length of the fixation lever arm (Fig. 16.8) but is appropriate when dorsal elements are not available for applying wires (e.g., following laminectomy).

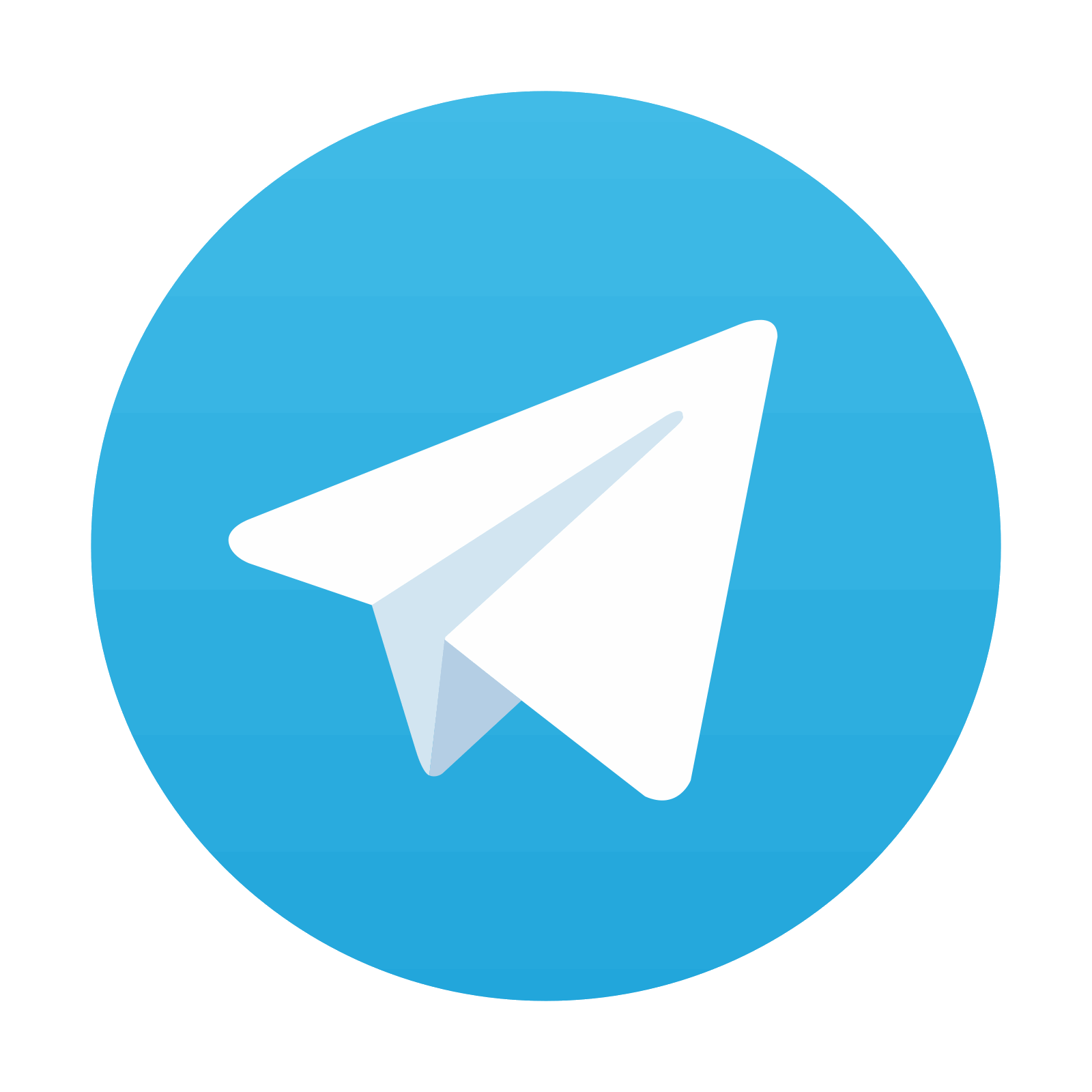
Stay updated, free articles. Join our Telegram channel
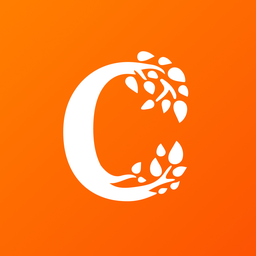
Full access? Get Clinical Tree
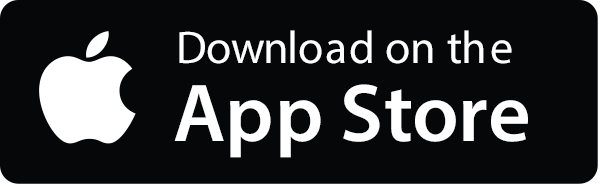
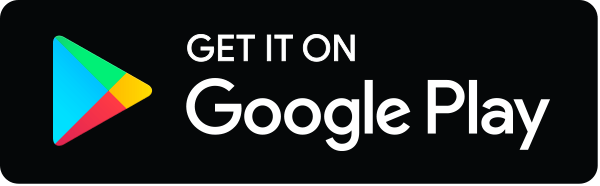
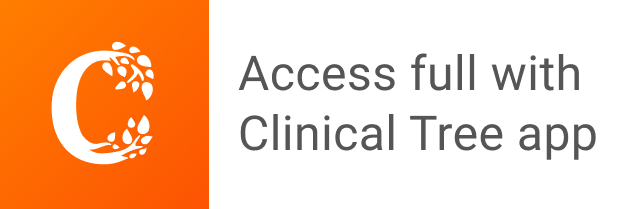