19 Construct Failure and Failure Prevention
Constructs can fail because of implant failure (e.g., fracture of a plate), failure of the bone at the implant–bone interface, or failure at a component–component juncture. 1 , 2 Each of these mechanisms of failure is addressed in this chapter.
19.1 Implant Failure
Implant failure is, in a sense, a poor choice of terms. Implants fracture and surgeons fail. Regardless, the term implant failure is used herein to describe the fracture of an implant or implant component or degradation of the interface between the implant and the “native” bone. Implants fail at points of maximum stress (θ) application. To be absolutely clear, implants always fail at the point of maximum stress application. Stress is a function of bending moment (M) and section modulus (Z). Stress (θ) is defined by the equation θ = M/Z, In which Z defines the ability of an object, such as a screw or rod, to resist bending. Z is proportional to the third power of the diameter of a rod or the third power of the inner diameter of a screw (see Chapter 2). Examples of two scenarios regarding fixed moment arm cantilever beam screws, one with a constant inner diameter and the other with a “ramped” (“tapered” or conical) inner diameter are portrayed in Fig. 19.1.
The point of failure of a fixed moment arm cantilever beam screw with a fixed inner diameter is usually at the screw–plate juncture (point of maximum stress application; Fig. 19.1a, b). The point of failure of a screw with a ramped (or tapered) inner diameter is somewhere between the tip of the screw and the plate, usually closer to the tip (Fig. 19.1c, d). 3 In the example depicted in Fig. 19.1, the bending moment increases linearly along the screw (dotted line) in both scenarios. The solid lines depict the stress (bending moment/section modulus, or M/Z), which is unchanged along the length of the screw for a fixed-inner-diameter screw but rises exponentially (to the third power) for a tapered-inner-diameter screw. Again, it cannot be emphasized enough that implants ALWAYS fail at the point of maximum stress application.

The longitudinal member (i.e., plate or rod) itself can fail by fracture. Long moment arms and bending moments that are applied to regions of an implant with a relatively small section modulus (Z) result in the application of significant stress (θ = M/Z). This has been shown to be the case with the Harrington distraction rod system (Zimmer; Warsaw, IN; Fig. 19.1e). In Fig. 19.1e, two versions of the Harrington distraction rod are portrayed: an 11-ratchet rod and a 7-ratchet rod. The 11-ratchet rod was removed from the market because of a high incidence of fracture at the lowest ratchet (not the case in this example), which was a consequence of the fact that hooks were commonly placed near the terminus at the first or second ratchet. Thus, the application of a long moment arm and bending moment to the most caudal or lowest ratchet resulted in a high incidence of fracture. Nonfixed moment arm cantilever beam screws (screws that toggle in the plate) also fail at points of maximum stress application. Nonfixed moment arm cantilever beam screws are often exposed to forces very different from those applied to their fixed moment arm counterparts. Fixed moment arm cantilever beam screws are exposed to fixed moment arm cantilevered moment arms and loads that are perpendicular to the long axis of the screw (see Fig. 19.1), along with their associated stresses. Nonfixed moment arm cantilever beam screws are exposed predominantly to three-point bending moment arms and loads, which also are oriented perpendicular to the long axis of the screw (Fig. 19.2a), potentially resulting in screw fracture (Fig. 19.2b). As an axial load is applied to a nonfixed moment arm cantilever beam screw–plate construct, the screw “sees” different force vectors (in both magnitude and orientation) at various points along the screw (Fig. 19.3). This can result in fracture (see Fig. 19.2b). These are usually three or four in number and relate both to the loads applied and to the varying consistencies and integrities of the materials through which the screw passes. The latter vary from the metal of a plate to cancellous bone and can cause a “shear” effect that results from a three- or four-point bending mode of load application. This results in a bending moment that stresses a fixed moment arm cantilever beam screw (almost always with a fixed inner diameter) maximally at the point of maximum bending moment application (see Fig. 19.2a, lower), which occurs at the point of intermediate force application where the bending moment is greatest. Such is more figuratively depicted in Fig. 19.3.


The toggling of a nonfixed moment arm cantilever beam screw in bone degrades the integrity of the bone, as well as the integrity of the screw–bone interface, by its “windshield-wiping” (sweeping) motion. It may result in the screw itself abutting the end of the bone graft, thus obliterating a portion of the surface area of contact between the bone graft and vertebral body (Fig. 19.4).

The strength characteristics of plates are defined, in part, by their section modulus (Z). With plates, the section modulus is a function of cross-sectional area and geometry. Although a plate may appear to be bulky, it is no stronger than its weakest link (Fig. 19.5). Like screws or rods, plates fail at the point of maximum stress application (θ = M/Z). This is the point at which the ratio of the applied bending moment to the section modulus (M/Z) is maximum (Fig. 19.6 ). 3 , 4 A plate, screw, or rod is most vulnerable at the point of maximum bending moment application if the section modulus is unchanged. If the section modulus (Z) is not adequate, fracture will occur (see Fig. 19.6).


Rigid (fixed moment arm) multisegmental constructs tend to load the caudal screws more than the rostral screws. This can cause failure of the caudal screws (Fig. 19.7a). Long fixed moment arm cantilever beam screw implants, in general, are associated with a relatively high failure rate. 5 In this situation, the use of a shorter implant (if clinically appropriate) is associated with the application of a shorter bending moment to the screws, thus decreasing the chance of failure (Fig. 19.7b, c). A corollary of this phenomenon is observed in the low cervical spine and the cervicothoracic junction, where the regional anatomy and geometry apply additional stresses to the caudal screw–bone interface (Fig. 19.7d, e). 6

Screw fracture may pose unique problems related to extraction. A variety of strategies have been employed for this purpose. 7 It should be remembered, though, that screw extraction may be difficult and is often not necessary.
19.2 Implant–Bone Interface Failure
Implant–bone interface failure can be obviated by not employing implants. 1 In the cervical spine, this has been shown to be relatively prudent in selected circumstances. 8 – 11 More modern clinical strategies, however, obviously may cause one to challenge this “philosophy.” Ultimately, decreasing motion by means of an implant has a positive effect on fusion. 4
The integrity of the implant–bone interface can be optimized in many ways. The ability of the implant to resist failure is in part related to its ability to distribute loads (load sharing), so that no single portion of the implant or spine bears an excessive portion of the load (load bearing). In other words, it is optimal if applied loads are distributed over multiple implant components and multiple points of contact with the spine (load sharing). This can be accomplished by (1) improving the integrity of the existing implant -bone interfaces, (2) providing additional implant -bone interfaces, (3) improving the integrity of the bone, and (4) normalizing geometry. 12 Additionally, any strategy that off-loads an implant obviously minimizes the chance of implant failure. This has been accomplished via the use of dynamic implants (see Chapter 29). Axially dynamic implants rarely fracture, thus lending further credence to the aforementioned notion that any strategy that off-loads the implant obviously minimizes the chance of implant failure. Axially dynamic implants, by the nature of their application, bear very little axial load. They substantially resist translation and angulation, but not axial loading.
19.2.1 Improving the Integrity of Existing Implant–Bone Interfaces
Screw geometry can be altered so that pullout resistance is enhanced (see Chapter 15). Similarly, the concept of triangulation can be used to a pullout resistance advantage (see Chapter 15). 12 – 14 Spine configuration must also be taken into account. For example, cervical spine lordosis causes screw–bone interfaces at the ventral cervicothoracic junction to be exposed to loads that may be associated with an increased chance of failure (see Chapter 29). 6
Additional innovative strategies include expanding the screw tip (e.g., like a molly bolt or drywall screw), buttressing the vertebral body margin (see the following), and augmenting the screw hole (e.g., with bone chips or polymethylmethacrylate; see Chapter 15 and below). An appreciation of the changing angular relationships of the lower cervical spine as the spine is descended, particularly at the cervicothoracic junction, is imperative (see Fig. 19.7d, e). These cause significant loading of the caudal screw–bone junction and can lead to failure, 6 especially at the caudal end of long ventral cervical constructs. However, it is also related to the angle of the screw in relation to the axis that is perpendicular to the floor (see Fig. 19.7d, e).
19.2.2 The Provision of Additional Implant–Bone Interfaces
The provision of additional fixation points provides a biomechanical advantage via two mechanisms: (1) the provision of additional points of fixation and (2) the provision of the ability to resist deformation in more than one plane and by more than one mechanism. Regarding the former, the integrity of fixation is proportional to the number of “high-quality” fixation points in “native bone” (load sharing). The ability to resist spinal deformation in more than one plane and by more than one mechanism is illustrated in Fig. 19.8 by the example of a rigid ventral construct acting as a fixed moment arm cantilever beam (Fig. 19.8a). In this situation, in which two screws are used at each terminus of the implant, axial loads are borne relatively well, in fact arguably equally as well as is observed with a single-level anterior cervical decompression fusion with plating (see Fig. 19.8a). However, implants and implant–bone interfaces rarely fail following simple one-dimensional loading. They usually fail following repetitive loading (fatigue) via multiple orientations. This has been shown to occur with regard to implants that are applied without intermediate points of fixation to the “native spine.” 15 The loads are usually applied from a variety of angles and orientations. These include transverse, rotational, and shear loads. The implant depicted in Fig. 19.8a bears axial loads well, as already stated. However, it does not effectively resist translational and rototational loads (Fig. 19.8b). This is so because the long moment arm associated with the long implant applies significant stress to the spine when such loads are applied. This may cause degradation of the screw–bone interface (Fig. 19.8 c), and ultimately failure, as a result of axial loading (Fig. 19.8d). The longer the implant, the more prone the implant becomes to these effects. The addition of intermediate points of fixation allows the implant to resist these translational loads via a third fixation point and three-point bending mechanical strategies (Fig. 19.8e). Thus, the additional fixation points not only permit axial loads to be borne more effectively but also increase the resistance to translation, rotational, and shear forces. This has been shown to be the case in the lumbar spine with the employment of pedicle screws. 16 Axial loads can be borne more effectively with additional intermediate fixation points into the “native spine,” as well. Consider the fact that most implants are flexible (Fig. 19.8f). The extent of deformation (bowing) of a flexible implant is significantly limited by the use of an intermediate fixation point (Fig. 19.8g). 16 These intermediate points of fixation must be to the “native spine.” Bone graft attachment sites provide little overall stability and in fact weaken the bone graft (Fig. 19.9). Stability is not enhanced by adding an intermediate point of fixation to a bony strut. In fact, stability is actually lessened. The flexibility-related bowing of the implant, as alluded to above, is not impeded by this strategy. Instead, it causes harmful ventral and dorsal loads to be applied to the strut that may increase motion at the mortise–strut interface by affixing the strut to the implant (see Chapter 29). Finally, in this regard, when an implant is not used with multiple-level discectomies, they are associated with a high failure rate via pseudarthrosis. 17 This observation further underscores the importance of multiple-level fixation to the “native bone.”


In the case of a long ventral corpectomy with decompression operation, attaining an intermediate point of fixation to the parent spine may be achieved in the cervical spine by “leaving” an intermediate vertebral body. For example, if one were to leave the C5 vertebral body intact for a C4–C6 decompression and a C3–C7 fusion, C5 could be used as an intermediate point of fixation, thus employing C3–C5 and C5–C7 interbody fusions instead of a single C3–C7 interbody strut–graft fusion (Fig. 19.10). This may (and probably does) offset the disadvantages associated with the increased number of fusion interfaces that must heal. Another method of achieving the same biomechanical advantage (i.e., intermediate points of fixation) is to employ a ventral and a dorsal operation (ventral corpectomies plus dorsal lateral mass fixation). 18 The lateral mass fixation points, however, are not as solid as an intermediate vertebral body. A second operation is also required, with its associated morbidity and even potential mortality. Nevertheless, corpectomies at three or more levels with strut–graft fusion may be effectively augmented by adding a dorsal implant. 19

Other fixation points include spikes (e.g., the tetra spikes of the Kaneda system [DePuy-AcroMed, Raynham, MA]) and a buttress point of fixation (e.g., using fins) at the end plate in the region of the cortical margin (Fig. 19.11). This takes advantage of the “boundary effect” whereby the edge of the end plate and the cortical wall of the vertebral body bear axial loads much more effectively than the interstices of the end plate and vertebral body.

The use of additional vertebral body screws (e.g., three instead of two) may provide a theoretical biomechanical advantage. However, the volume of bone displaced by the metal and its effect on bony purchase and bone integrity must also be considered (Fig. 19.12a, b).

Of particular note regarding the majority of spine fixation strategies is the emphasis on the importance of optimizing each individual fixation point. For example, the surgeon must ensure that optimal implant–bone contact is achieved, lest suboptimal implant–bone interface contact result. The careful crafting and contouring of the implant “seat” on the vertebral body is critical (Fig. 19.13a, b).

Intermediate points of fixation may also be provided by the use of the interference screw technique (see Chapter 37). Passing a screw between an interbody strut graft and the “native” vertebral body remnant at the margin of the trough following the performance of a cervical corpectomy may provide a surface for solid contact of the screw with the parent vertebral body. Both the strut and the trough can be “gripped” by the interference screw. An interference screw is simply a screw that captures two separate, but juxtaposed, bony surfaces. In this case, it captures the bone graft and the remnant of the “native” vertebral body at the medial border of the corpectomy trough. Although the former provides little support via “capture” of the bone graft, the latter may provide a significant advantage regarding an intermediate point of solid fixation with the “native” spine. Furthermore, the bone graft may be forced or wedged against the contralateral (to the interference screw) corpectomy wall, thus augmenting stability (Fig. 19.14).

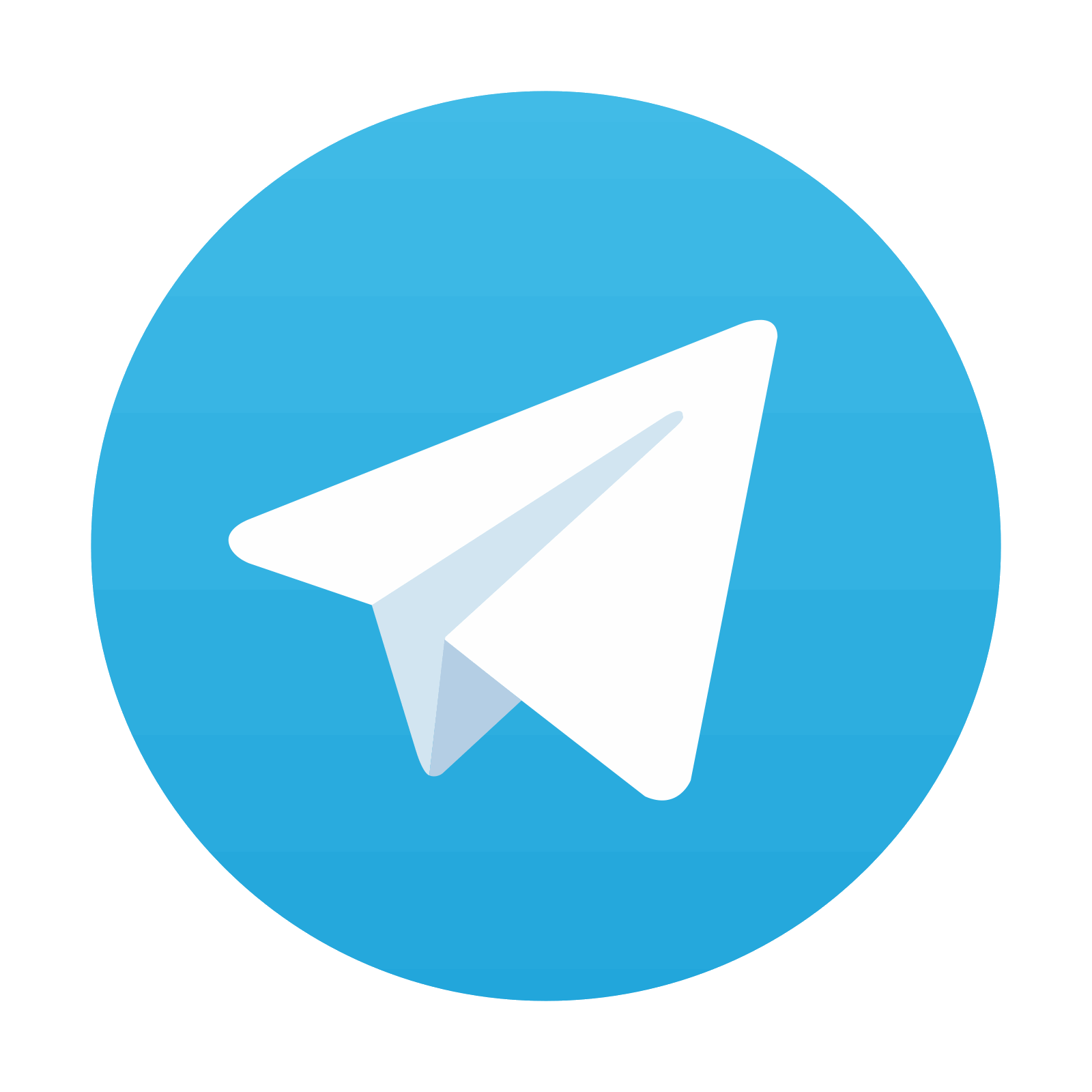
Stay updated, free articles. Join our Telegram channel
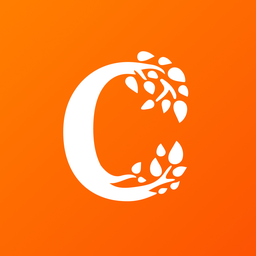
Full access? Get Clinical Tree
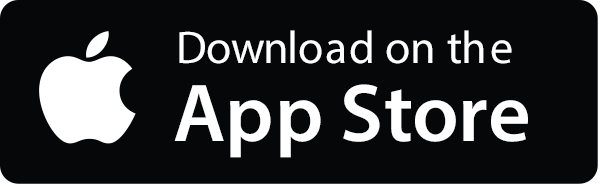
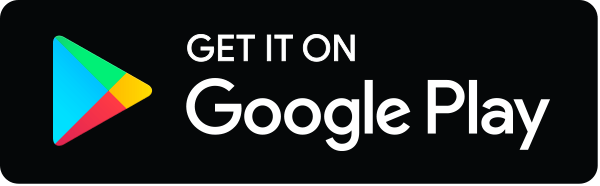
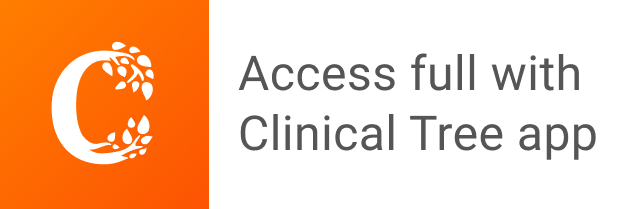