2 THEORETICAL BASIS OF FLOW DIVERSION
Abstract
Flow-diverting devices induce a mechanical disruption of flow into and out of aneurysms which then induces physiological phenomena which lead to aneurysm thrombosis and endothelial overgrowth. Ultimately, these processes culminate in the anatomical reconstruction of the parent artery. These processes are directly affected by a complex interaction between the physical properties of the implanted flow diverter, the geometry of the parent artery–aneurysm complex, and the status of the coagulation system. We examine the existing data describing these interactions in reference to clinical practice.
2.1 Introduction
Flow diverters are fine-mesh stents that are implanted in cerebral arteries at the site of aneurysms. They are so named because their primary function is to “divert” blood flow away from the aneurysm into the parent artery (strictly speaking, they reduce flow into and out of the aneurysm). The theoretical sequence of phenomena governing flow diversion treatment of aneurysms can be listed as follows: (1) the placement of a fine-mesh screen at the aneurysm neck reduces blood flow through the aneurysm, (2) the sluggish flow promotes clot formation within the aneurysm, (3) the fine-mesh screen is paved over by a new arterial wall lining, and (4) the thrombosed aneurysm is resorbed by the body′s wound healing mechanisms—the end result of which is a remodeled vessel returned to its normal physiological state. These events are schematically described in Fig. 2.1. While it is a relatively straightforward list, needless to mention, the actual interplay of the various processes involved is quite complex. This chapter gives a brief overview of the therapeutic mechanism of flow diversion.

2.2 Device Mesh Structure
The mesh structure of flow diverters is crucial to their effective function; therefore, at the very least, a flow diverter′s mesh needs to be far finer than that of a stent ( Fig. 2.2a). Because the device acts as a porous screen at the aneurysm neck, the characteristics of this porous media are defined by the mesh structure. Porous media are studied in a wide variety of fields, such as hydrogeology, petroleum engineering, ceramics, concrete, and textiles. In general, these fields evaluate two fundamental characteristics of the medium—porosity and pore connectivity—to assess the overall permeability. 1 , 2 , 3 While permeability is dependent on the porosity measure, it does not rely only on this variable. 3 For flow diverters, the two variables effecting device permeability and, by extension, device treatment efficacy can be termed as porosity and pore density. Porosity is the percentage ratio of the metal-free surface area (total surface area–metal surface area) to the total surface area. This is alternately referred to as metal coverage (ratio of metal surface area to total surface area). Pore density is number of pores per unit surface area of the device. Flow diverters are manufactured by braiding (helically wrapping multiple metal wires into a tubular structure) and the fundamental variables governing device design are the device diameter, the wire diameter, and the angle that the wires make to the device axis (called braiding angle). The nominal or design porosity and pore density of a device can be mathematically expressed in terms of these variables.

Using these mathematical expressions, theoretical variations in porosity and pore density of a characteristic flow diverter as it is deployed in arteries of varying diameter can be plotted ( Fig. 2.2b). Braiding allows for the device wires to slip on each other and thus elongate longitudinally when deployed in arteries with smaller diameter than the device′s in-air diameter and, vice versa, expand in diameter when compressed longitudinally (such device “packing” at the aneurysm neck section is common practice when deploying some commercial flow diverters). While this feature allows for superior wall apposition in cerebral vessels of varying diameter, the deployed pore structure of a braided device, and hence its permeability at the aneurysm neck, will vary depending on parent vessel diameter, curvature, aneurysm neck size, etc. ( Fig. 2.2c). As the plots show, device oversizing can cause a maximal change (relative to designed values) in porosity and pore density of around 20 to 25% for most braided flow diverters. Whether this range effects aneurysm treatment efficacy is not yet clear, but a preliminary study 4 suggests that just a 3% absolute difference (~ 12% relative difference) in deployed device porosity can result in different aneurysm outcomes. The point of minimal efficacy (highest porosity and lower pore density) occurs when the angle between the wires is 90 degrees. On the other hand, radial expansion of the devices by longitudinal compression results in decreased device porosity and increased pore density.
2.3 Intra-aneurysmal Flow Alterations
Detailed evaluations of the changes in intra-aneurysmal hemodynamics due to flow diverters have mostly been conducted via in vitro and numerical studies. 5 , 6 , 7 , 8 , 9 , 10 , 11 The traditional or theoretical view of flow patterns in untreated idealized sidewall aneurysms holds that the parent artery flow divides or impinges at the distal neck of the aneurysm, follows the distal wall to the dome, and exits the aneurysm near the proximal neck ( Fig. 2.3a). This forms a vortex within the aneurysm that rotates opposite to the direction of flow in the parent vessel. Given the complexity and variability of patient aneurysm geometries, this flow pattern can vary substantially with the pulsatility and strength of the parent artery flow, aneurysm geometry and its orientation with respect to the parent vessel, and the presence of side branches at or near the aneurysm neck. Depending on these parameters, for example, there may be a single vortex with its center undulating (with the cardiac cycle) within the aneurysm and transiently entering the parent vessel, 5 , 6 or the flow may divide after it enters the aneurysm and form regions with both clockwise and counterclockwise flow. 7 , 12 In general, and based mostly on numerical simulations, peak velocities ranging from 10 to 150% of the parent vessel velocity and average wall shear stress (shearing force per unit area exerted by fluid motion along the wall) values ranging from 10 to 220 dynes/sq.cm have been reported within aneurysms. 13

The placement of a flow diverter across the aneurysm neck breaks up the in- and out-flow patterns. Intra-aneurysmal flow activity (optimally) becomes restricted to the neck region ( Fig. 2.3a, Pipeline device) and is generally in the direction of parent artery flow, entering near the proximal neck and exiting near the distal neck. 5 , 7 , 14 , 15 Most flow studies thus far have involved sparse, low sample sizes because of the computational expense required to numerically simulate flow fields in flow diverted aneurysms or the device expense involved with benchtop studies. Several intra-aneurysmal hemodynamic parameters have been evaluated, but the most common ones are related to flow velocity or its derivatives (inflow rate, average velocity, maximum velocity, kinetic energy [proportional to velocity squared], vorticity, and hydrodynamic circulation [velocity measures of rotational/vortical motion]); wall shear stress, a function of velocity gradient and viscosity, is another common parameter. Aggregating the results from about 20 flow studies on idealized, in vivo, and patient geometries (87 patient cases, half of which are from two studies 10 , 11 ) suggests a spectrum of flow diversion response. Flow diversion reduces intra-aneurysmal flow activity in idealized/simplified sidewall-type geometries by about 75 to 95%, 7 , 16 , 17 , 18 , 19 in simplified bifurcation-type geometries by about 20 to 40%, 16 , 20 and in an in vivo aneurysm model by around 60%. 21 , 22 Reduction in intra-aneurysmal flow activity in clinical cases runs the entire gamut from around 20 to 30% in bifurcation geometries to around 80 to 90% in sidewall geometries with an average reduction of around 50 to 60%. 9 , 10 , 11 , 23 , 24 , 25 , 26 , 27 , 28 , 29 , 30
While this class of studies measure detailed intra-aneurysmal velocity fields with the concomitant experimental or computational expense associated with trying to simulate reality, another category 31 , 32 , 33 , 34 evaluates the intra-aneurysmal transport of angiographic contrast from the images already being acquired during the endovascular procedure; these studies also note significant increases in contrast residence time measures after flow diversion. As a side consequence of this sluggish flow after flow diversion, intra-aneurysmal blood viscosity increases, especially near the aneurysm dome. Because blood is a non-Newtonian fluid, its viscosity increases with decreasing shear rate 35 (velocity gradient or change in velocity over a distance). A numerical simulation considering non-Newtonian effects in a simplified geometry suggests a threefold increase in blood viscosity in more than half of the aneurysm sac as compared to the nonstented case. 36 Another noteworthy phenomenon is that there is essentially no change in intra-aneurysmal pressure due to the deployment of a flow diverter. 37
2.3.1 Effect of Pore Structure
The pore structure of a flow diverter primarily controls device-induced intra-aneurysmal flow alterations. As can be expected, reducing device porosity can substantially reduce flow activity within the aneurysm as has been confirmed by numerous studies over the past two decades. 6 , 7 , 16 , 18 , 20 , 27 , 38 , 39 The numerical simulation results ( Fig. 2.3a) show an example of dampened flow activity within a simplified sidewall aneurysm from the deployment of devices with decreasing porosity. The vortical flow structure (and velocity magnitude) is slightly reduced after the deployment of a high-porosity stent (Neuroform), but is severely diminished after deployment of a flow diverter (Pipeline). Fig. 2.3b shows the collated results from a few studies evaluating the effect of porosity on aneurysmal velocities. While decreasing porosity clearly reduces flow activity, there is generally a wide spread in the degree of reduction depending on the geometry of the aneurysm–parent vessel complex. Flow diverter–equivalent porosities can induce near-stagnant flows in sidewall and/or low flow aneurysms, while the flow reductions in bifurcation and/or high-flow patient geometries are comparatively less. Increasing pore density of device while maintaining a constant porosity also results in reduced intra-aneurysmal flow activity. 7 , 14 , 39 , 40 , 41 Again, there is a wide variation in the results thus far and a very loose extrapolation from these studies on simplified geometries suggests that doubling the pore density (at constant porosity) can result in reduction of flow activity ranging anywhere between 20 and 150%.
It is unclear whether a “one-size-fits-all” porosity or pore density threshold exists that will result in successful occlusion of all aneurysms, but given that 1-year aneurysm occlusion rates after flow diversion are reasonably high (~ 90%), porosities and pore densities of current commercial devices can be considered to be more or less in the correct range. Needless to mention, any theoretical considerations of minimizing porosity and/or maximizing pore density need to be balanced against practical considerations such as additional metal-to-artery burden resulting in in-stent thrombosis, increased device stiffness resulting in difficulties with navigating tortuous vessels, as well as coverage of side-branch ostia resulting in perforator occlusions. As shown in Fig. 2.1, the physiological pressure gradient across perforators jailed by flow diverters maintains flow through these side branches and the rate of perforator infarction is thus only about 3%. 42 This is also supported by results from flow studies that have evaluated flow through side branches 7 , 17 , 43 , 44 , 45 ; at maximum, flow diverters reduced the mean flow rate through the studied branches by 20%.
While the practice of “packing” flow diverter wires together at the aneurysm neck by longitudinal compression can theoretically improve flow diversion effects, there are precautions that must be considered during device deployment. In general, a uniform distribution of the pore structure covering the aneurysm neck will provide the most favorable flow diversion behavior. Marked differences in the pore structure can occur 46 and cause flow to preferentially enter the aneurysm through device segments with looser, more open pores and potentially lead to unfavorable results. The worst of these scenarios occurs when devices are mal-apposed to the arterial wall at the aneurysm neck resulting in a “jet”-type flow entry into the aneurysm from the gap between the device and the wall. 10 , 39 While more significant with stents, 20 flow diverters deployed across aneurysms at the outer curvature of vessels can have their pores spread open leading to increased flow activity within the aneurysm. A similar effect can occur when devices bulge into the aneurysm during device packing, causing pore structures to open up and increase intra-aneurysmal flow activity, 47 thus counteracting any beneficial effects of packing.
2.3.2 Predicting Aneurysm Occlusion
The primary goal of all these studies measuring intra-aneurysmal flow alterations is to derive flow-related parameters that can predict long-term aneurysm occlusion after flow diversion. If successful, such studies could facilitate optimal device selection prior to treatment and (the angiographic studies) could help modulate the treatment by assessing device efficacy in near-real time during the procedure. For example, Fig. 2.4 shows device-induced flow alterations in two right carotid aneurysms; the aneurysm with the 99% kinetic energy (KE) reduction was completely occluded at 6 months of follow-up, while the one with 52% KE reduction still had minimal residual filling at 6 months of follow-up. Sample sizes are, again, sparse but both in vivo 21 , 22 , 31 , 48 and clinical studies 9 , 10 , 23 , 49 , 50 , 51 have noted that the reduction in intra-aneurysmal flow activity (based on whichever variable the study chose to quantify) after flow diversion can be significantly different between aneurysms that remained patent at follow-up versus those that occluded at follow-up. Unfortunately, each research group chooses, even prefers, to define their own variable to define flow activity, and until some standard variables are evaluated by all laboratories on their patient datasets, it may be difficult to establish the index (indices) that is (are) able to predict long-term aneurysm occlusion after flow diversion. Such standardization will be required of both dominant paths of evaluation—angiographic analysis and numerical simulations—to establish a predictive index. Data are, however, being collected; for example, an aggregate of 95 patient cases (60 angiographic analysis 50 , 51 and 35 numerical simulations 10 , 23 ) suggests a reasonably high predictive value of chosen indices, with specificity and sensitivity of around 75 to 90%.

While these results are promising, whether or not a purely flow-derived parameter will be able to predict aneurysm occlusion without the consideration of biological/biochemical parameters is unclear at this point. The incorporation of biochemical factors into flow studies is in the incipient stages. 52 , 53
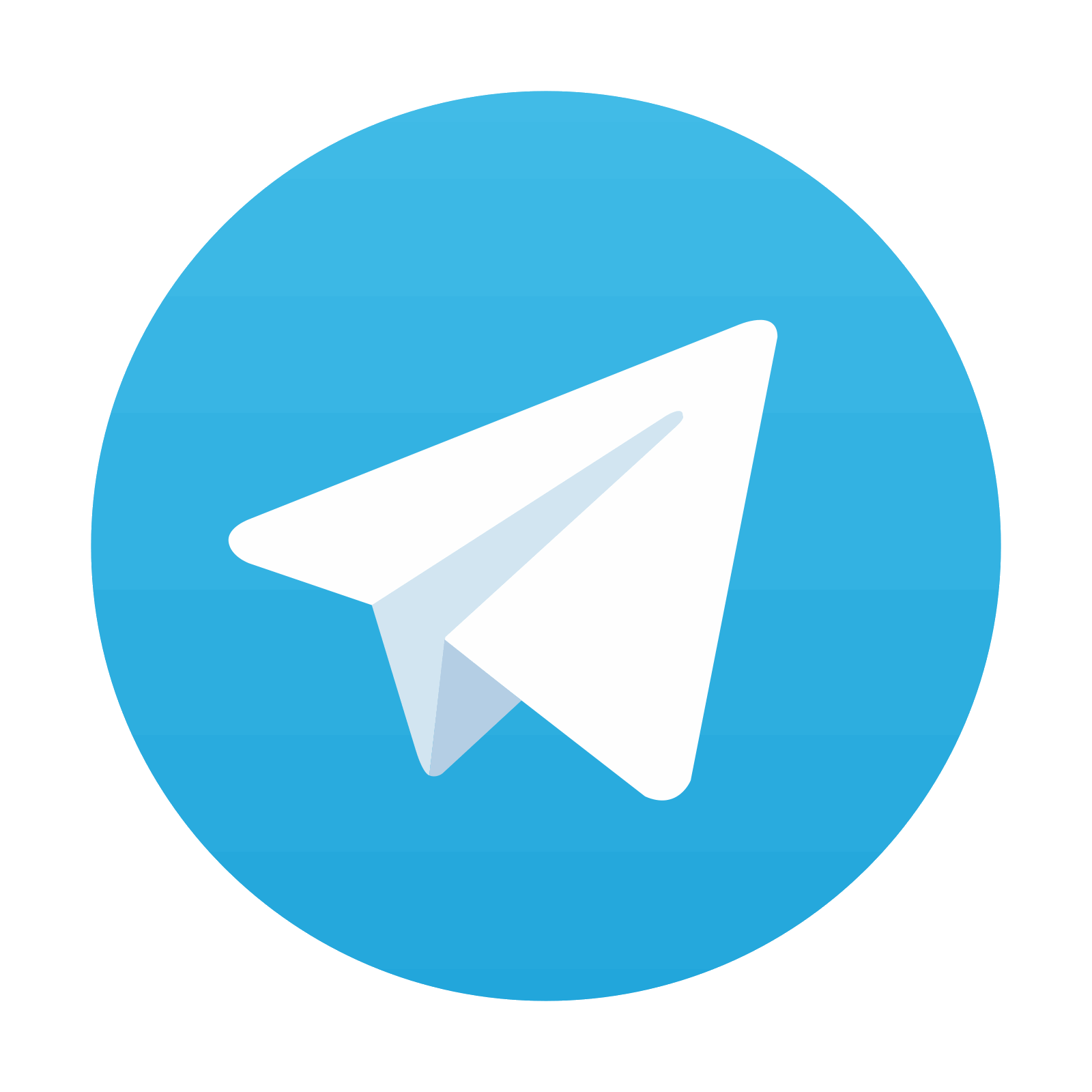
Stay updated, free articles. Join our Telegram channel
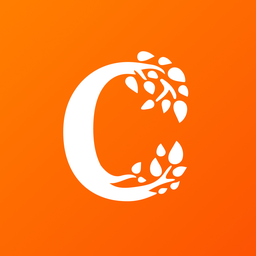
Full access? Get Clinical Tree
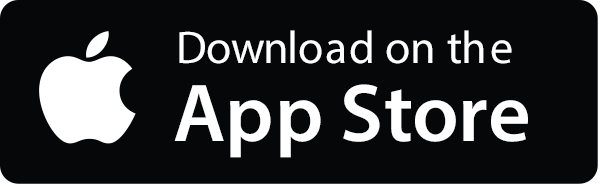
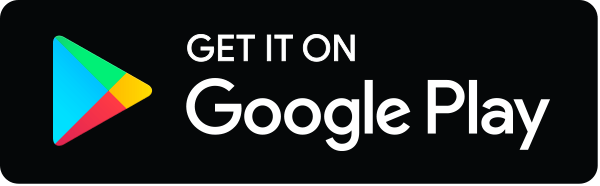
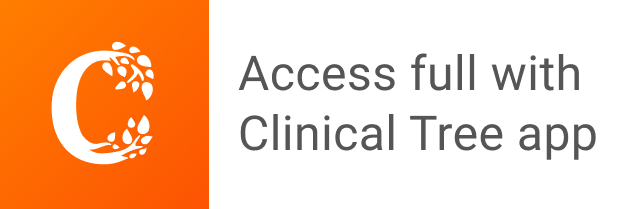