21 Radiotherapy for Pineal, Thalamic, and Brainstem Tumors
Abstract
The literature supporting the use of radiotherapy in the treatment of pineal, thalamic, and brainstem tumors is discussed in this chapter. An overview of the modalities of radiation delivery is provided, including external-beam radiotherapy, intensity-modulated radiotherapy, and proton beam radiotherapy. The special technical considerations of radiation delivery to pineal, thalamic, and brainstem tumors are reviewed, including organs at risk and dose constraints. Finally, the management of acute and long-term toxicities is addressed.
Biology of Radiotherapy
Introduction to Medical Radiation
Radiation has been used for more than 100 years to treat cancer, since shortly after the X-ray was discovered by Röntgen in 1895. 1 Many types of radiation are used in medical practice, including manufactured radiation and radiation from natural isotopes (e.g., iridium, iodine, and cesium). 2 The vast majority of radiation given for central nervous system (CNS) malignancies is delivered via manufactured X-rays. X-rays are produced in a machine (most commonly a linear accelerator [linac]). that accelerates electrons to a high energy and stops them in a target of tungsten or gold, thus producing high-energy X-rays. 1 X-rays can be thought of as “packets” of energy comprising photons; the shorter the wavelength of the X-ray, the higher the energy. 1 Linacs have the ability to deliver high doses of radiation to precise locations in the body, thus minimizing damage to normal tissues—this is termed external beam radiotherapy (EBRT). 2 For linac-based radiotherapy to the CNS, the beam energy is typically 6 to 10 MV. Conversely, radiation emitted from natural isotopes is predominantly used for brachytherapy, with a radioactive source placed directly into the body (e.g., into the resection cavity during intraoperative radiotherapy).
Although the majority of medical radiation involves the use of electromagnetic radiation (e.g., X-rays), it is important to note the emerging use of particulate radiation in the treatment of CNS malignancies. In particular, protons are positively charged particles with a mass nearly 2,000 times greater than that of electrons. 1 Given the size of protons, they require a cyclotron to be accelerated for therapeutic purposes and are favored for many CNS malignancies because of their favorable dose distribution.
Radiobiology
The cytotoxic effects of radiation result primarily from damage to DNA. 3 Radiation can either directly damage DNA (direct action) or interact with other molecules in the cell, especially water, to produce free radicals that subsequently damage DNA (indirect action) ( Fig. 21.1 1 ). Approximately two-thirds of the damage caused by X-rays is via indirect action, and this damage has the potential to be mitigated with the use of free-radical scavengers. For heavy particles such as protons, a higher proportion of DNA damage occurs via direct action. Radiation is thought to damage DNA via multiple mechanisms and, in particular, radiation has been shown to induce single- or double-strand DNA breaks, induce base damage, induce abnormal crosslinks between DNA strands or between proteins and DNA, and induce chromosome aberrations. 2 , 3

Radiosensitivity describes the effects of radiation on cells and is influenced by the degree of regression of tumor cells, how quickly they regress, and the duration of response. 2 After exposure to radiation, cells might die during the next mitosis (mitotic death) or via programmed cell death (apoptosis). 4 A cell’s DNA repair mechanisms influence its radiosensitivity: unlike normal cells, malignant cells are thought to be preferentially destroyed by low-dose radiation because of differences in DNA repair capabilities. After receiving a low dose of radiation (e.g., 1–2 Gy), tumor cells and normal cells sustain sublethal damage to their DNA. Normal cells can repair this sublethal damage relatively quickly compared to tumor cells. Thus, radiation is typically administered in a fractionated schedule (low doses every day) because this schedule gives normal cells a chance to repair themselves while malignant cells accrue lethal damage.
Overview of Radiation Delivery
The majority of radiation for CNS malignancies is delivered via EBRT whereby radiation from an outside source is aimed at the body and delivered to the target. As radiation traverses the body, a dose is deposited in all tissues (malignant and nonmalignant) along the path. This section describes how radiation is delivered to maximize dose to the target and minimize the unavoidable normal-tissue toxicity.
Treatment Planning
Treatment planning for radiotherapy consists of developing a plan for radiation delivery. The first step in treatment planning consists of patients undergoing a “simulation” or “mapping” scan that is typically a computed tomogram taken with consideration for the patient’s unique treatment position. The radiation target is defined by first outlining gross tumor volume, which should include all visible disease. The clinical target volume is then outlined such that the target is expanded to include areas of presumed microscopic disease spread. The clinical tumor volume depends on the known natural history of the tumor being treated as well as anatomical boundaries. Finally, the target is expanded uniformly into a planning target volume, which accounts for daily setup uncertainty. For brain tumors, a magnetic resonance image (MRI) is often fused to the computed tomogram to facilitate target delineation. For patients undergoing postoperative radiotherapy, the operative report is carefully reviewed with the surgeon to fully delineate areas at risk for microscopic disease.
After the targets are defined, dosimetry consists of determining the number of radiation beams to use, the angle of beam entry, and the shape and size of treatment blocks in three dimensions with the goal of conforming dose around the target and minimizing normal-tissue toxicity. Dosimetry is an iterative process whereby the delivery of radiation dose is optimized such that the target is covered generally with 95 to 100% of the prescribed dose, and critical nearby structures do not receive what is considered to be an unacceptable toxic dose. All treatment plans are unique and depend on the size and shape of the target in the context of an individual’s unique anatomy. To maximize dose to the target and minimize dose to uninvolved brain parenchyma, radiation oncologists employ three-dimensional (3D) conformal radiotherapy (3D-CRT) in which multiple beams deliver radiation converging on the target. For targets with unfavorable geometry or proximity to critical structures, intensity-modulated radiotherapy (IMRT) is a sophisticated form of 3D-CRT in which optimization software creates fluence patterns of virtual beamlets to achieve greater conformity for irregular shapes.
Fractionation Schedules
Radiation is typically given in a “fractionated” fashion, with low doses administered every day. The number of fractions corresponds to the number of radiation treatments delivered for a given treatment course. The ability of radiation to control or eradicate a tumor depends on the total dose delivered as well as the dose delivered per fraction. A higher dose per fraction results in more cell kill for both normal and malignant cells. Lower doses of radiation delivered over time allow for repair of normal tissues and have the potential to decrease late radiation effects on normal tissue. However, lower doses may allow for malignant cell regeneration between treatments, and therefore the cumulative treatment dose must be higher. The total therapeutic dose is calculated by multiplying the dose per fraction by the number of fractions.
Standard Fractionated External Beam Radiotherapy
The traditional fractionated dose of EBRT is 1.8 to 2 Gy per fraction. As described in this chapter, the total doses typically used for the treatment of pineal, thalamic, and brainstem gliomas are in the range of 45 to 66 Gy. When SRS is delivered in 1.8 to 2 Gy per fraction, a total of 25 to 33 treatments is required to deliver the total therapeutic dose. Radiation is typically delivered Monday through Friday with weekend treatment breaks incorporated to minimize toxicity.
Stereotactic Radiosurgery
Stereotactic radiosurgery (SRS) involves the delivery of a high dose per fraction in one to five fractions with rigid immobilization and precise image guidance to achieve submillimeter precision. The term SRS specifically refers to treatment delivered in a single fraction. Doses for intracranial SRS range from 12 to 35 Gy in a single fraction, depending on the histology, size of target, proximity to critical structures, and goals of care. SRS is the primary focus of Chapter 20 (“Stereotactic Radiosurgery for Tumors of the Brainstem, Thalamus, and Pineal Region”).
Several commercially available specialized radiation delivery systems have been developed to deliver SRS, including the Gamma Knife (Elekta) and the CyberKnife (Accuray). The Gamma Knife uses a hemispheric distribution of cobalt-60 sources that are precisely coordinated to converge at the target. Patients wear a metal collimator helmet when receiving treatment with the Gamma Knife. The CyberKnife uses radiation generated from a small linac with a robotic arm that allows the radiation to be delivered to the target from any direction. Patients wear a mask for head immobilization, but the CyberKnife also incorporates skull-base tracking methods to account for any movement of the target during treatment.
Indications for Standard Fractionated EBRT versus SRS
The choice of standard fractionated EBRT versus SRS depends on a balance between safety and convenience. Fractionating the total dose into multiple low doses per fraction treatment affords greater safety and a wider therapeutic window with which to deliver a sufficient dose to the tumor while sparing nearby critical organs. SRS can be much more convenient than EBRT, but its use is limited by tumor target size and proximity to critical structures, which mandate careful patient selection. In general, standard fractioned EBRT is necessary for larger targets (>3 cm) and for targets that are near critical structures such as the brainstem, optic chiasm, and optic nerves. SRS can be used for smaller targets that are, in general, more than 5 to 10 mm away from critical structures.
Proton Beam Radiotherapy
Given the importance of minimizing normal-tissue toxicity associated with radiation for CNS malignancies, there has been substantial interest in the last 50 years in developing proton beam radiotherapy. Protons are positively charged heavy particles that have an advantage over photon therapy because protons can deliver an equivalent dose to the target with a minimal exit dose (i.e., the dose delivered to normal tissues beyond the target). Traditional photon beams or electron beams deposit most of their energy near the surface with a smaller dose in deeper tissues. Conversely, protons deposit a lower dose near the surface and leave most of their energy in the final millimeters of their trajectory. Tissues beyond the targeted tumor receive very little dose with proton beam therapy. The sharp localized dose is known as the Bragg peak. Compiling beams of successively lower energies and intensities creates a “spread-out Bragg peak” that is able to deliver the intended dose to a 3D target. 5
Where it is available, proton therapy is a popular choice for the treatment of CNS malignancies, especially in pediatric populations ( Fig. 21.2 6 ). Proton therapy has the dosimetric advantage of minimizing normal-tissue toxicity and the theoretical clinical advantages of minimizing risk of late neurocognitive and neuroendocrine deficits and second malignancies. 7

Dose Limitations: Organs at Risk
The location of pineal, thalamic, and brainstem tumors implies that in nearly all cases critical normal tissues will receive considerable radiation dose. The complications associated with radiation treatment arise as a function of the toxicity induced by radiation to nearby structures. The Quantitative Analyses of Normal Tissue Effects in the Clinic (QUANTEC) guidelines 8 serve as an important benchmark for normal-tissue toxicity by establishing important dose limits for the brain, optic nerves and optic chiasm, brainstem, spinal cord, and cochlea. 9 , 10 , 11 , 12 This chapter focuses on normal-tissue toxicity in the setting of standard fractionated EBRT because it is important to understand that a different set of dose constraints applies to SRS, and these constraints are discussed in Chapter 20.
A feared complication of CNS radiation is radionecrosis. The risk for radionecrosis is highest in the first 2 years after standard fractionated radiation. 13 Symptomatic patients may exhibit nonspecific symptoms such as seizures, intracranial hypertension, and focal neurologic deficit. Unfortunately, radionecrosis is difficult to distinguish from tumor progression on MRI, but some data show that positron emission tomography or single-photon emission computed tomography can be used to differentiate hypometabolic radionecrosis from hypermetabolic tumor progression. 13 The risk of radionecrosis is less than 3% at a maximum dose of less than 60 Gy to the brain, but it increases to 5% with a maximum dose of 72 Gy and to 10% or greater with a maximum dose of 90 Gy. 14
Particular attention is given to minimizing the risk of brainstem injury. The maximum dose to the brainstem should be kept at less than 54 to 64 Gy to keep the risk of brainstem neuropathy or necrosis at less than 5%. 10 , 14 If the volume of brainstem receiving 59 Gy or less is kept between 1 and 10 cm3, then the risk of neuropathy or necrosis is believed to be less than 5%. 14 The risk of radiation-associated spinal cord myelopathy is estimated at 0.2% for a maximum dose of 50 Gy, 6% for a maximum dose of 60 Gy, and 50% for a maximum dose of 69 Gy. 12 , 14 Thus, 45 Gy is considered a conservative maximum dose of radiation for the spinal cord.
Radiation-induced optic neuropathy is estimated to occur with maximum doses of less than 55 Gy at a rate of less than 3%. 11 , 14 The risk of optic neuropathy increases to 3 to 7% with maximum doses of 55 to 60 Gy, and is estimated to be 7 to 20% at maximum doses of greater than 60 Gy. Hearing loss is estimated to occur with a frequency of less than 30% at a maximum dose of 45 Gy to the cochlea. 9 , 14 Given these toxicities, careful treatment planning is necessary to ensure safe radiation delivery.
Finally, it is important to note that frequently radiation is given concurrently with radiosensitizing chemotherapy. Since chemotherapy increases the expected toxicity of radiation, safe dose limits are likely lower than when radiation is delivered without chemotherapy.
Pineal Tumors
Overview
The pineal gland is located on the posterior wall of the third ventricle in the quadrigeminal cistern, surrounded by the splenium of the corpus callosum superiorly, the thalamus anteriorly, and the quadrigeminal plate and vermis inferiorly. 15 The parenchyma comprises pinealocytes and supportive astrocytes. 16 Although Descartes viewed the pineal gland as the seat of the soul, we now know that pinealocytes secrete melatonin, which is a hormone involved in the circadian rhythm. 17 Pineal tumors are rare and comprise 1% of primary CNS tumors. 15
Patients with pineal tumors can present clinically with signs of increased intracranial pressure (e.g., nausea, vomiting, and headache) secondary to hydrocephalus because pineal tumors can obstruct the sylvian aqueduct, preventing cerebrospinal (CSF) flow from the third to the fourth ventricle. Parinaud syndrome (i.e., inability to gaze upward, convergence-retraction nystagmus, and impaired pupillary reaction to light and accommodation) is a classic presentation of patients with pineal tumors compressing the superior colliculus. 16 Compression of the periaqueductal gray matter can lead to mydriasis, convergence spasm, and pupillary inequality. Endocrine abnormalities, particularly precocious puberty and diabetes insipidus, can develop in patients with pineal tumors. 16 , 17
Pineal tumors exhibit a wide histopathologic heterogeneity due to the variety of structures that become neoplastic in the pineal region. 15 , 16 Embryonal remnants give rise to germ cell tumors, the ependymal layer gives rise to ependymomas, and the choroid plexus gives rise to choroid plexus papillomas. 15 Furthermore, the subcommissural organ of the third ventricle can give rise to papillary tumors of the pineal region (PTPR), the velum interpositum can transform into a meningioma, and the deep venous system can produce vascular malformations. Germ cell tumors are the most common tumor involving the pineal gland (35%), followed by glial tumors (including ependymomas and PTPR, 32%), and tumors of pineal parenchymal cells (28%). 15 , 18
Germ Cell Tumors
Germ cell tumors typically affect adolescents and account for 3 to 5% of childhood brain tumors. 19 These tumors typically occur in the pineal gland or suprasellar region. There is a male predilection for pineal gland tumors (5:1), whereas suprasellar tumors occur at equal frequency in males and females. Among germ cell tumors, 10% are bifocal. Germ cell tumors are divided into two prognostic histologic groups: germinomas, which have the most favorable prognosis, and nongerminomatous germ cell tumors (NGGCTs), which have a less favorable prognosis. 19 It is important to differentiate between pure germinomas and NGGCTs because this distinction has a profound impact on prognosis and treatment recommendations. Patients with a new diagnosis of a germ cell tumor should receive complete staging with contrast-enhanced MRI of the brain and spine, serum and CSF beta-human chorionic gonadotropin and alpha-fetoprotein tests, and CSF cytology. CSF should be obtained by lumbar puncture if this can be safely performed.
Germinomas
Radiotherapy is a standard component of care for patients with pure germinomas. 16 , 20 Historically, germinomas were treated with craniospinal irradiation (CSI) alone. 19 CSI involves treating the entire brain and spinal column to 18 to 36 Gy, which is then followed by a boost to the primary tumor to 50 to 54 Gy ( Fig. 21.3 ). Durable control can be achieved with CSI (> 90%) 21 ; however, CSI is associated with significant long-term neurocognitive and neuroendocrine adverse effects. 19 MAKEI 83/86/89 was a prospective, nonrandomized study undertaken to investigate dose reduction in 60 patients with germinomas. 22 In this study, CSI to 30 Gy with a 15-Gy boost was compared with CSI to 36 Gy with a 14-Gy boost. Complete remission (CR) was achieved in all patients, and the 5-year relapse-free survival was 91% at a mean follow-up of 59 months.

For most patients with localized germinomas, whole-ventricular system radiotherapy (WVRT) has replaced the use of CSI ( Fig. 21.4 ). Rogers et al 23 pooled outcomes from a large series of 788 germinoma patients compiled from 20 studies and found that relapse rates increased with smaller radiation volumes: 4% after CSI, 8% after whole-brain radiotherapy (WBRT) or WVRT, and 23% after focal treatment alone. Isolated spinal relapse was not different between CSI and WBRT/WVRT groups (1.2% vs 2.9%) but was significantly higher in the limited field/focal radiation group at 11%. Therefore, delivery of radiation to the whole ventricular system followed by a boost to the tumor bed has replaced CSI for localized germinomas. 19 In the SFOP (Société Française d’Oncologie Pédiatrique) analysis of pattern of failure and outcome of 60 patients with nonmetastatic germinomas treated with chemotherapy and limited-field radiation, 16% of the cohort developed intraventricular relapse, illustrating the importance of utilizing WVRT in the initial treatment of localized germinoma. 24 WVRT is necessary given the propensity of germinomas to spread along the ventricles. Proton therapy can be used in WVRT to reduce radiation of healthy tissues without compromising dose to the target compared with photon radiation given via 3D-CRT or IMRT modalities. 19

To reduce the late adverse effects of radiotherapy for localized germinomas, pediatric oncologists favor the use of chemotherapy (most commonly carboplatin-etoposide for two to four cycles) followed by reduced dose/volume radiation. 25 The most standard regimen for localized germinomas is chemotherapy followed by 21 Gy to the whole ventricles, followed by a boost to the tumor to a total dose of at least 30 Gy in 1.5-Gy fractions. The ongoing Children’s Oncology Group (COG) trial (ACNS1123) is investigating the safety of reducing the total radiation dose to 18 Gy to the whole ventricles, with a 12-Gy boost to the tumor in 123 patients with localized germinomas who exhibit a complete or partial response to four cycles of induction chemotherapy with carboplatin and etoposide. CSI is given only to patients with CSF-positive disease or metastatic disease. It is important to note that bifocal disease is considered to be localized disease.
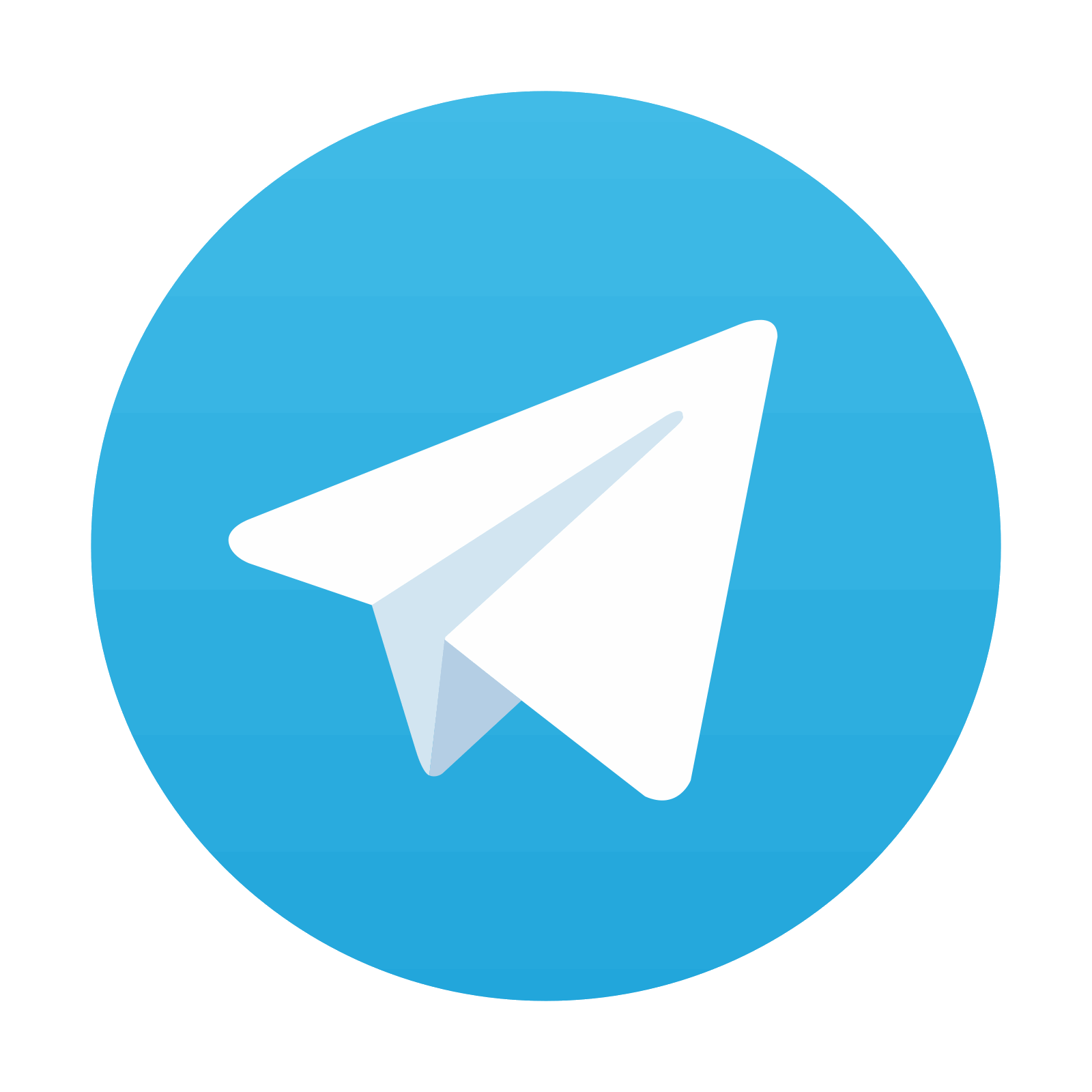
Stay updated, free articles. Join our Telegram channel
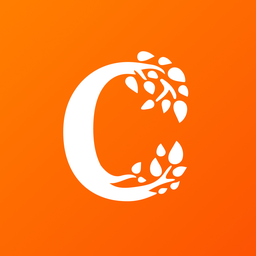
Full access? Get Clinical Tree
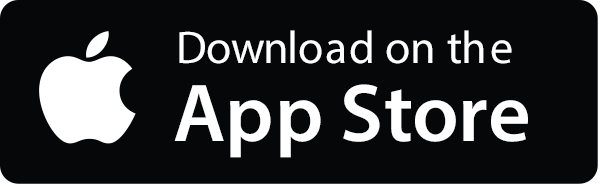
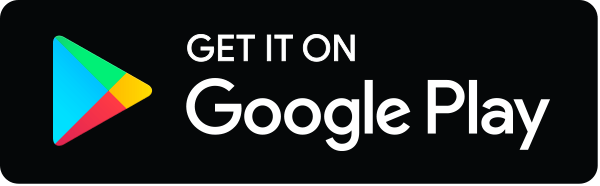
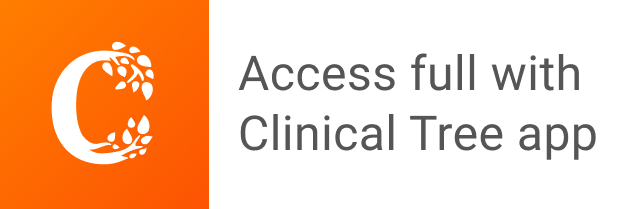