25 Osteobiological Agents for Spinal Fusion
Complications in harvesting autogenous iliac crest bone-graft material, including pain at the donor site, has led to the investigation of potential alternatives to such grafts, including various allograft sources, demineralized bone-graft material, and bone morphogenetic protein (BMP). This chapter will review both alternative options and the biology involved in spinal fusion.
Fracture Healing
For the treating surgeon to understand the process of spinal fusion, it is necessary to understand the process of healing of a bone fracture. Such healing involves the interaction with one another, in a well-orchestrated sequence of events, of four major types of tissue: cortical bone, periosteum, external soft-tissue sleeve, and bone marrow. Rigid internal fixation leads to primary cortical healing, which involves a biological response with remodeling units of cutting cones consisting of osteoclasts, followed by an influx of osteoblasts.1 This type of healing is typified by an absence of bony callus. When rigid internal fixation is not provided to support healing, micromotion occurs at the fracture site. In this latter case, healing involves a combination of intramembranous and endochondral bone formation, in which both the periosteum and the external soft tissues are involved. This type of healing is typified by the formation of bony callus.
Intramembranous Ossification
Intramembranous bone formation occurs without a cartilaginous intermediate. In the fracture callus, new bone is formed by osteoblasts located adjacent to the fracture site and deep to the proliferating periosteal cells. Immediately after injury, positive staining for BMP is evident in the cambium-cell layer of periosteum proximal and distal to the fracture site. As healing of the fracture progresses, the number of these periosteal cells increases. By 1 week after fracture there is evidence of new woven bone formation, with abundant staining of osteoblasts lining this primitive bone. As the amount of intramembranous bone increases over the course of the next few days, there is a corresponding increase in the number of osteoblasts lining the woven bone. As the process of lamellar bone formation progresses, the overall numbers of periosteal cells decrease as do the percentage of cells staining positively for BMP ( Figs. 25.1 , 25.2 , 25.3 , 25.4 , 25.5 , 25.6 ). As the process of intramembranous bone formation proceeds and the number of more mature cell types increases, the presence of BMP decreases.2,3






Endochondral Ossification
To study the endochondral healing of a fracture, a closed transverse femoral fracture has been established in a rat model.4 This type of fracture is associated with minimal soft-tissue damage, and is characterized by several different fracture-healing responses. The first 7 to 10 days of fracture healing involve the process of chondrogenesis, which leads to cartilage formation adjacent to the fracture site and the formation of bone directly from osteoprogenitor cells under the periosteum. An inflammatory response occurs at the fracture site, heralded by the presence of macrophages, polymorphonuclear leukocytes, and lymphocytes, which secrete proinflammatory mediators. By 14 days after fracture, discrete areas within the cartilage anlage begin to calcify. In preparation for calcification, the chondrocytes release phosphatases and proteases. The phosphatases provide phosphate ions that combine with and precipitate with the calcium delivered from the mitochondria to form calcified cartilage. The proteases work by degrading inhibitory proteoglycans, allowing the chondrocytes to control the rate of the mineralization process.4 In this model, the mid-diaphyseal fracture is well on its way to being united by 3 weeks after fracture. The callus is composed mainly of calcified cartilage, which is ultimately removed and replaced by bone. This process is initiated by chondroclasts, which are multinucleated cells specialized in the resorption of calcified tissues. The chondroclasts mediate vascularization and allow the arrival of mesenchymal stem cells that differentiate into osteoprogenitor cells and, ultimately, into bone-forming osteoblasts. The removal of calcified cartilage includes not only resorption of the mineralized matrix but also removal of the chondrocytes themselves. By 4 to 5 weeks after fracture, a combination of calcified cartilage and newly formed woven bone is present at the site of injury. At this time, large numbers of osteoclasts populate the tissue and begin to remodel the callus, converting it to a lamellar bone structure capable of supporting mechanical loads. This transition from cartilage to bone after injury involves a highly organized series of cell and molecular events leading to cell removal and matrix modification.4
Although the exact molecular basis for fracture healing is still unclear, several protein growth factors and cytokines are known to play an important role in the process of skeletal tissue repair.5–8 Members of the transforming growth factor-β (TGF-β) supergene family, which include the BMP genes, have been shown to control several processes during skeletogenesis and repair. Cho et al 9 described the temporal expression of members of the TGF-β supergene family during fracture healing in a mouse-tibia model over a 28-day period. Within 24 hours after the fracture was sustained, there was an increase and a peak in activity of BMP-2. By the day 7 there was maximal expression of other cartilage genes: growth and differentiation factor (GDF)-5, TGF-β2, and TGF-β3. In contrast, BMP-3, BMP-4, BMP-7, and BMP-8 showed a restricted period of expression during the central period of the fracture-healing process from day 14 through day 21, when the resorption of calcified cartilage and osteoblastic recruitment were most active.
The Biology of Spinal Fusion
The osterolateral arthrodesis of lumbar intertransverse processes is the most common spinal-fusion procedure, yet failure to achieve a solid bony union occurs in as many as 10 to 40% of patients with single-level uninstrumented fusions of this type. The percentage of such failure is even higher when multilevel fusions are attempted.10 This high rate of nonunion indicates that the success of any fusion operation depends on a complex interplay of numerous physiological, biological, and molecular events. The most common recent clinical approach to preventing nonunion in the posterolateral spine has been the use of internal fixation with a pedicle-screw-rod construct. Although the use of internal fixation has decreased the number of nonunions, it has not eliminated the problem. Nonunions still occur in 10 to 15% of patients with instrumented fusions. Therefore, studying the biological sequence of events in spinal fusion provides key insights into devising biological products and bone-graft substitutes to enhance fusion rates.10
The biology of spinal fusion is a multifactorial process, which makes it difficult to study in the clinical setting. In the past, the fusion rates in animal models used to investigate the biological events in the healing of a fusion approached 100%, a figure much higher than is seen clinically. These models were skeletally immature organisms, and the fusion done in them was either an interfacet or an interlaminar fusion rather than a truly intertransverse fusion. In contrast to spinal fusions in humans, these fusions in animal models were usually unsuccessful only if the model involved a destabilized spine. These limitations led to the development by Boden et al of a rabbit model of intertransverse-process arthrodesis that was more clinically applicable to the human situation.11 The major benefit of this type of model was that in it, nonunions occurred spontaneously at a rate comparable with that reported in humans who had fusion without internal fixation.
The rabbit model of lumbar intertransverse-process arthrodesis has been well characterized with the use of autogenous iliac crest as the graft material. Mechanically solid fusions in the model generally occur by 4 to 6 weeks, with an overall rate of nonunion of 30 to 40%. Radiographic analysis has shown progressive remodeling of bone graft material with time, usually by 10 to 12 weeks, but as in humans, radiographs were accurate in assessing success or failure to attain solid fusion only 70% of the time. Control animals showed that surgical exposure alone did not automatically result in spinal fusion, as has also been seen in other species.11 Vascular injection studies indicated that the primary blood supply to the fusion mass originated from the decorticated transverse processes. The failure to achieve spinal fusion in the absence of decortication emphasizes the importance of careful fusion-bed preparation of the posterolateral elements of the spine for successful fusion. Proper preparation of the fusion bed is required to provide critical bone marrow, vascularization, and cellular elements to the fusion mass.11
Three distinct and reproducible temporal phases of healing in spinal fusion have been histologically identified, including an inflammatory phase, an endochondral phase, and a remodeling phase.12 Histological analysis indicated that the maturation of a fusion occurs from the periphery and progresses centrally. The maturation of a spinal fusion was most advanced at the ends of the fusion mass, near the transverse processes (outer zone). A similar histological progression occurred in the central zone, but was delayed relative to the series of events in the outer zones. A similar lag has been noted in osteoblast-related gene expression, with the peak expression of all genes occurring 1 to 2 weeks later in the central zone than in the two outer zones. This is consistent with the peripheral-to-central healing pattern observed histologically in fusions done with autogenous bone-graft material. This central lag effect, with a transient cartilaginous area, was hypothesized as the reason for many nonunions in the central zones of fusion masses.
Variations were also seen in the temporal and spatial expression of the mRNA for BMP. In the peripheral zones, expression of the mRNA for BMP-2 was increased during weeks 2 through 6, with peak expression at weeks 3 and 4. BMP-6 in the outer zones had a first peak on day 2 and a second peak during week 5. BMP-6 in the central zone showed an initial peak on day 2, but did not demonstrate a later peak. This lower level of expression of BMP-6 observed in the central zone of the fusion mass may be correlated with the delayed timing and smaller amount of bone formation in the central zone ( Figs. 25.7 , 25.8 , 25.9 ).12
The use of electrocautery in the healing of spinal fusion is controversial. Some spine surgeons use electrocautery (Bovie dissection) sparingly or avoid it completely through fear of increasing the chances of postoperative infection and delaying wound healing. They prefer to ligate bleeding vessels and mechanically elevate the muscles from the bone subperiosteally. However, most surgeons use Bovie dissection extensively. They feel that it is is quicker and produces much less bleeding during surgery. There is no evidence in the literature that extensive use of electrocautery alters cytokine expression or impairs the progression of fusion. We do not feel that extensive electrocautery in any way contributes to psuedarthrosis after spine surgery.


Osteobiological Products and Spinal Fusion
Nearly half a million bone-graft procedures are performed in the United States annually. Of these, the vast majority are spinal fusions, potentially representing up to $2 billion spent per year on agents for enhancing bone repair and bone-graft substitutes ( Table 25.1 ).13,14 Because of the distinct biological conditions and biomechanical forces specific to the anterior spinal column and the posterior elements, it is likely that the efficacy of each bone-graft material in promoting successful fusion will also depend on the particular clinical application for which it is being used (e.g., interbody fusion versus posterolateral fusion) ( Table 25.2 ).15–17

The processes of bone regeneration and formation during spinal fusion require three critical elements: (1) osteogenic cells that have the capacity to make new bone; (2) osteoinductive factors (i.e., growth factors and cytokines) that promote the differentiation of stem cells into an osteoblastic phenotype; (3) and an osteoconductive scaffold that facilitates neovascularization and supports the ingrowth of bone. Autogenous bone is believed to have all three of these essential properties for bone formation. It is therefore considered the “gold-standard” graft material for spinal fusion. Autograft bone for use in the spinal column is most often taken from the iliac crest, but may also be obtained from other sources, such as a resected rib or from local bone harvested at the fusion site, depending on the location and extent of the surgical procedure ( Table 25.3 ). Procurement of autogenous bone often necessitates a separate operative incision, thus involving additional iatrogenically induced surgical trauma. Besides increasing the operative time and blood loss, the harvesting of bone-graft material from a separate site is also associated with considerable donor site morbidity. Nearly 30% of patients who undergo such procedures experience some kind of complication postoperatively. The complications include infection, hematoma, nerve or vascular injury, fracture, persistent pain, abdominal herniation, and pelvic instability. The amount of bone available for grafting may also be insufficient in children as well as in adults requiring revision surgery or fusion of multiple spinal segments.18–20 Given the limitations of autogenous bone grafts, significant research has been done to find and improve graft-extending materials and other sources of grafts.
A bone-graft extender is a substance that adds bulk to a given amount of autogenous bone that is to be used over a greater surface area with the same rate of success. A bone-graft enhancer is a substance that when added to autograft bone increases its healing potential with either the usual or a smaller amount of graft material. A bone-graft substitute is a substance that may entirely replace autogenous bone-graft material with the same or a better rate of successful fusion.21
Allograft Bone
Allograft bone obtained from cadaveric sources has been the most widely used substitute for autogenous bone-graft material.22 Allografts are osteoconductive, with minimal or no osteoinductive potential, primarily because the donor’ s cells are eradicated during tissue processing. Allografts are prepared either by freezing or lyophilization (i.e., freeze-drying) to decrease their antigenicity and permit their storage for extended periods. Frozen allografts may be kept for up to 1 year at -20°C without a change in their structural properties. Lyophilized allografts are dehydrated and vacuum packed, which allows their storage at room temperature. Freeze-drying reduces the immunogenicity of allografts more than does freezing, but upon rehydration and reconstitution, freeze-dried grafts may lose up to 50% of their mechanical strength. Allografts may also be treated with ethylene oxide or radiation, although these methods may further compromise their material properties and reduce their osteoinductive capacity.22
A common patient concern with the use of cadaveric allografts is the possible spread of infectious diseases, such as hepatitis and human immunodeficiency virus (HIV) infection. To date, only two cases of transmission of HIV in allograft bone have been documented, both of which involved unprocessed grafts. Only one of these cases involved allograft bone used for a spinal fusion. The combination of meticulous donor screening and tissue processing has reduced the risk of infection from allograft bone to less than one per million transplants.23
Cortical allografts offer substantial structural stability and are well suited for inter-vertebral-body arthrodesis. Threaded cylinders (of cortical allogratft) may serve as a source of bone-graft material while stabilizing the spinal column. Hollow centers allow the insertion of carrier soaked in growth factors or autograft material to aid anterior fusions. Fusion with these techniques occurs slowly, by means of periosteal new-bone formation around the allograft. Cortical allografts never fully incorporate at their site of engrafting, and remain a mixture of necrotic and viable bone. On the other hand, corticocancellous allograft material initially imparts very little mechanical support, but because of its relatively large surface area is integrated more rapidly and fully than a purely cortical bone graft.
Both autogenous bone and allograft bone are incorporated into the fusion mass according to a well-defined cascade of biological events, consisting of hemorrhage, inflammation, and vascular invasion, culminating in the replacement of graft material with new bone. With bone allograft this remodeling process occurs more slowly and there is greater resorption of the graft than with autograft bone. This may manifest itself in the inter-vertebral-body milieu by cortical lucencies that indicate resorption and vascular invasion. Genetic incompatibility of the donor and recipient has been found to be associated with increased resorption of allograft bone and histological evidence of rejection; however, routine screening for genetic compatibility may be impractical.24
The differing mechanical and biological properties of the various types of allografts make each type suitable for differing applications. Predominantly cortical allografts, such as femoral rings or fibular struts, can be useful in applications in which there is a need for structural support under compression (e.g., inter-vertebral-body applications). Allografts of cancellous bone, such as cancellous-bone chips, are best suited in areas that require little mechanical strength and greater osteopromotion such as posterolateral fusions. In these applications there is a need for more rapid bone incorporation, remodeling, and revascularization.
Zdeblick and Ducker25 obtained equivalent results when using allograft or autograft bone for single-level fusions, but the incidence of nonunion was 62% among patients receiving allograft bone for two-level anterior cervical fusion, as compared with only 17% among patients receiving autologous bone. It must be noted, however, that these results were acieved before the advent of routine anterior cervical plating. In another retrospective review of patients treated with multilevel uninstrumented arthrodeses of the cervical spine,26 An and colleagues found that fusion occurred in 85% of those implanted with autograft bone, whereas successful healing occurred in only 50% of a group treated with allografts. These results were contested by Samartzis et al,27 who reviewed the fusion and clinical outcome data for 80 consecutive patients who underwent anterior cervical discectomy and fusion (ACDF) with either allograft or autograft bone and anterior plate fixation involving two and three vertebral levels. Of these 80 patients, 45 received autogenous tricortical grafts from the iliac crest and 35 received tricortical allograft bone and were treated with multilevel ACDF with anterior plate fixation at a single institution. The overall rate of fusion was 97.5%, with no significant difference in the rates of fusion with allograft and autografts. Excellent and good clinical outcomes were noted in 88.8% of the patients. The literature appears to support the success of single-level noninstrumented fusions with autograft bone, but in biomechanically challenging multilevel fusions, the results with the use of rigid instrumentation are the same with allograft or autograft bone.
In two studies, patients treated with autograft were found to have solid posterolateral fusion more often than those receiving allograft bone.28,29 Interestingly, Dodd et al30 reported a 100% fusion rate in adolescent patients with idiopathic scoliosis who had implants of femoral head allograft and local autograft bone. Betz and colleagues31 compared the clinical results of posterior spinal fusion (PSF) with allograft-bone augmentation and those without grafting in patients with adolescent idiopathic scoliosis (AIS). Ninety-one patients with AIS were randomized into two treatment groups. Seventy-six patients had more than 2-years of follow-up and were included in this review. In the group treated with allograft bone alone, 37 patients underwent a standard PSF with multisegmented hook-screw-and-rod instrumentation with the use of corticocancellous bone allografts for augmentation. The study group included 39 patients with AIS who underwent the same procedure without any bone graft (no graft group). The treatment groups were similar with respect to age, preoperative deformity, and degree of postoperative correction. The overall pseudarthrosis rate was 1.3%. Pseudarthrosis was identified in a single patient in the study. This pseudarthrosis occurred in the allograft group, whereas none of 39 patients in the no-graft group experienced pseudarthrosis. Betz and colleagues concluded that PSF with newer-generation multisegmented hook-screw-and-rod systems could be successful with allograft or local bone graft or both without the use of supplemental autogenous bone graft in patients with AIS. In summary, these studies suggest that corticocancellous allografts may be used as either graft extenders or as alternatives to autogenous bone grafts in many spinal-fusion procedures.
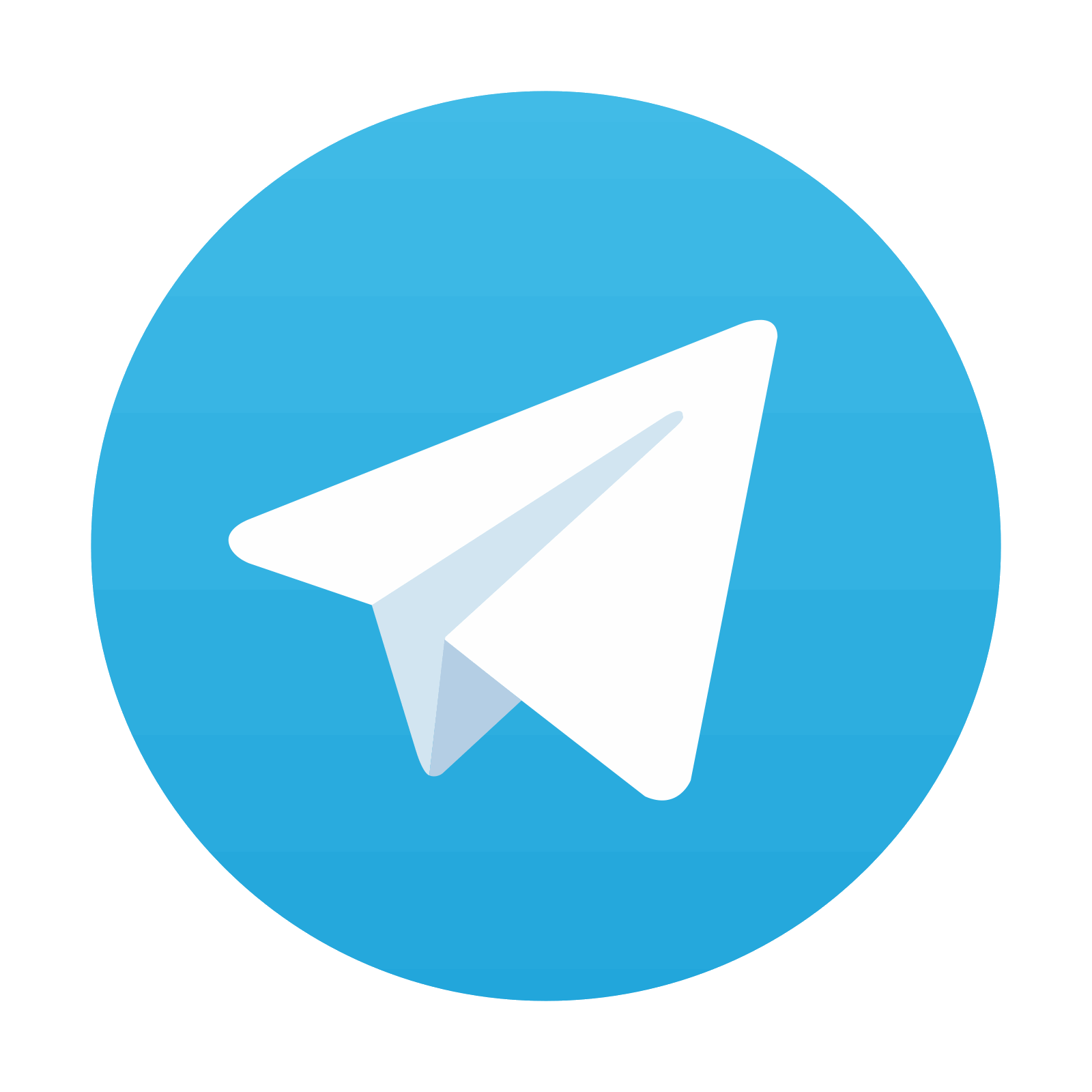
Stay updated, free articles. Join our Telegram channel
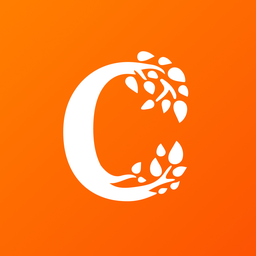
Full access? Get Clinical Tree
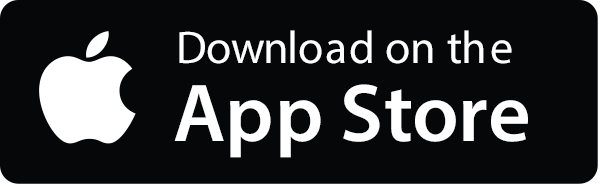
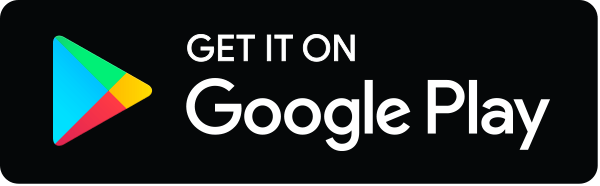
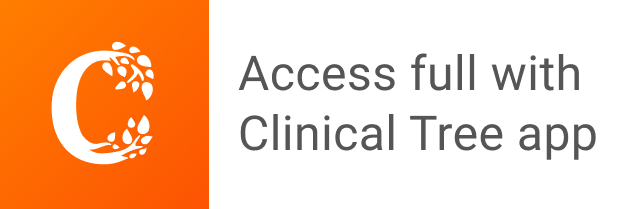