28 Complex Instrumentation Constructs and Force Applications
Most, if not all, spinal implants are complex. For the purposes of this chapter, however, complex spinal implants are considered to be those that employ multiple and/or complex force applications. The complexity of instrumentation techniques varies depending on the region of the spine and the biases and opinions of the surgeon. Biases and opinions are not often altered. Therefore, a region-specific approach, for the most part, is undertaken in this chapter.
28.1 Craniocervical Region
A variety of techniques have been used to stabilize the craniocervical region. As outlined in Chapter 1, the craniocervical region (the occiput, the occipital condyles, and the C1 and C2 vertebrae) is associated with complex movements and load resistance mechanisms. At the occiput–C1 segment, flexion occurs with very little rotation or lateral bending. At the C1–C2 segment, minimal lateral bending, moderate flexion and extension, and significant axial rotation occur (see Chapter 1). To put it simply, the occiput–C1 joint functions predominantly in flexion and extension, whereas the C1–C2 joint functions primarily in rotation and secondarily in flexion and extension (Fig. 28.1). It is imperative, therefore, that flexion–extension, lateral bending, and axial rotation be restricted by craniocervical instrumentation techniques.

The occiput provides several options for implant fixation. However, the depth of bone to which screws can be anchored is short, except in the midline. In addition, the risks associated with screw fixation are not insignificant. Therefore, many surgeons used cable–rod techniques in days gone by (Fig. 28.2), whereas most now use midline occipital screw fixation techniques. However, the latter resist rotation poorly because of the relatively long moment arm applied to the screws (Fig. 28.3). Laterally placed occipital screws may be used to minimize the rotatory tendency (Fig. 28.4a). Cross fixation may compensate somewhat by creating a quadrilateral frame construct (Fig. 28.4b). Regardless, they do not provide as much fixation potential as midline screws (Fig. 28.4c). A plate that is fixed to the occiput by midline screws and that securely abuts the occipital bone laterally on both sides perhaps provides the optimal occipital bone fixation (Fig. 28.4d). Cables or wires may cut through the thin bone of the occiput. Hooks pose similar dilemmas, as well as technical problems. Pait and colleagues introduced a unique occipital fixation approach, the occipital button technique, which was moderately popular for a short time (Fig. 28.5). 1 Regardless, techniques such as the occipital button technique underscore the importance of lateral fixation, which minimizes the rotational instability associated with motion about the long axis of the spine (see Fig. 28.3).




Often, occipitocervical fixation is complicated by the need for long caudal fixation lever arms; hence, it is often necessary to employ a fixation strategy that aggressively incorporates the middle to lower cervical spine. If rigid or solid occipital fixation is achieved, the subaxial component of the construct may indeed be the “weakest link” of said construct (see Chapter 20 and Fig. 28.6).

The surgeon must have reasonable expectations of any implant and should consider much more than simply the axial load-bearing potential of an implant. The prevention of translational and rotational deformations is also extremely important, and it should be remembered that implants function differently under differing loading conditions. Unique and varying forces are resisted by the implant in each of these loading situations (circumstances). Considering the upper cervical spine as akin to a universal-like joint with a ball and socket component may assist the surgeon in understanding the complexities of upper cervical spine motion and the loads, forces, and moments that must be resisted (Fig. 28.7). 2

Rotatory deformation at the C1–C2 segmental level (see Fig. 28.1 and Fig. 28.7) may complicate occiput–C1 stabilization procedures by permitting the relatively unrestricted C1–C2 rotation to weaken the occiput–C2 fixation construct. The elimination of this rotational deformation (i.e., by rigid C1–2 fixation; Fig. 28.8a) may permit the use of shorter constructs by providing a substantial platform for occiput–C1–C2 fixation (Fig. 28.8b, c). Otherwise, extension of the construct to C3 or below may be in order (Fig. 28.8d).

28.1.1 High Cervical Region
The Gallie, Brooks, and combination techniques have been used for C1–C2 fusions for decades (Fig. 28.9). 3 – 5 All (especially the Gallie technique) minimally resist sagittal plane translation deformation. This deformation occurs in a parallelogram-like manner (Fig. 28.10). Because the C1–C2 facet joints are axially oriented with respect to each other in the sagittal plane, they minimally contribute to parallelogram deformation resistance (see Chapter 22). Nevertheless, the Brooks and combination techniques 3 , 4 provide a level of augmented rigidity that the Gallie technique does not. The combination technique, described by Sonntag and colleagues, provides a biomechanical advantage equivalent to that of the Brooks technique without its singularly most significant disadvantage, the risk associated with bilaminar sublaminar wire passage. 4


C1–C2 clamp techniques, such as the Halifax clamp, 6 provide a safety factor with respect to the lack of need for sublaminar wire passage. However, they are prone to the same translation deformation complications as the aforementioned wiring techniques (Fig. 28.11).

28.1.2 Three-Point Bending Screw Techniques
Three-point bending screw techniques may be applied in any region of the spine. For years they have been used for translaminar and facet fixation in the lumbar region (Fig. 28.12). 7 – 10 In recent years, however, they have been used mainly in the upper cervical spine, ventrally for the fixation of dens fractures (Fig. 28.13) 11 and dorsally for C1–C2 transarticular fixation to manage C1–C2 instability (Fig. 28.14). 2 They use and withstand a combination of complex forces, the predominant force complex application being three-point bending (Fig. 28.15a, b). Because the screws resist three-point bending loads, their ability to resist failure (fracture) is exponentially (to the third power) related to their inner diameter. Although a cannulated screw is weaker than an equivalent noncannulated screw, this effect is usually minimal (Fig. 28.15c, d). It is emphasized that these implants are usually placed in a neutral mode at the time of surgery. However, they are required to resist three-point bending forces during the activities of daily living. These forces are oriented perpendicular to the long axis of the screw.




An understanding of the loads to be withstood by such constructs is essential for their appropriate use. Both screw size and moment arm length should be carefully considered. For example, a three-point bending force application to a thin cantilevered screw may be appropriate if the transverse load is not great and the moment arm is not long. If these conditions are exceeded, a larger screw or a shorter moment arm should be considered (Fig. 28.16).

28.2 Subaxial Spine
The subaxial spine is defined as the entire spine below the axis (C2). Of note, the lumbosacral region is regarded as a separate and distinct region because of its terminal position in the spine and the complex and unique forces and loads to which it is exposed. The lumbosacral region, henceforth, is addressed as a unique region of the spine, as was the craniocervical region in the prior section of this chapter. Therefore, it is discussed separately in the next section rather than in the present section, “Subaxial Spine.”
28.2.1 Cervical Spine
With regard to complex cervical fixation, the mechanisms by which ventral implants fail is of significant relevance. Subsidence and a failure to effectively resist transverse (e.g., three-point bending) loads are the predominant mechanical contributors to construct failure (see Chapters 19 and 29 and Fig. 28.17). The failure of long constructs is particularly problematic (Fig. 28.18). 12 Using an axially dynamic implant may play a role in complication reduction in selected cases by off-loading the implant and increasing bone healing–enhancing forces (Wolff’s Law; see Chapter 29 andFig. 28.19). The provision of adequate load-bearing capability is a necessity for the effective employment of interbody techniques. This has been commonly associated with the ability to resist subsidence. The end plate, however, may have been given undeserved credit for this ability. 13



As mentioned previously, transverse loads can be more effectively resisted if an intermediate point of spine fixation is provided (see Chapter 19). This (1) increases the number of spine fixation points, (2) enhances the ability to bear axial loads via a cantilever beam mechanism, and (3) provides an ability to resist loads applied perpendicular to the long axis of the spine. Regarding the latter mechanism, the ability to provide or resist three-point bending forces minimizes the chance that an implant will work loose during repetitive transverse load bearing via degradation of the screw–bone interface. Such a technique is depicted in Fig. 28.20. This strategy may be used to minimize or eliminate the need for combined ventral and dorsal procedures in selected situations, particularly cervical spine applications. When poor bone quality is a factor, strategies that improve the quality of the screw–bone interface, such as expandable tip screws, may be employed. 14 , 15

Dorsal cervical strategic decision making is often a straightforward process. One can apply creative techniques to enhance stability, when such is deemed necessary. This is exemplified by the combination of two techniques (i.e., a tension-band fixation technique with a cantilever beam technique—“belt and suspenders”; Fig. 28.21). In situations in which stability and subsequent spine deformation are of much less concern, a laminoplasty, or even laminectomy alone, may be appropriate. These have been shown to be biomechanically sound. 16 Careful patient selection is, however, mandatory.

The cervicothoracic junction poses unique challenges. Strategies that facilitate combining dorsal cervical and dorsal thoracic techniques are currently few in number. One such strategy is depicted in Fig. 28.22.

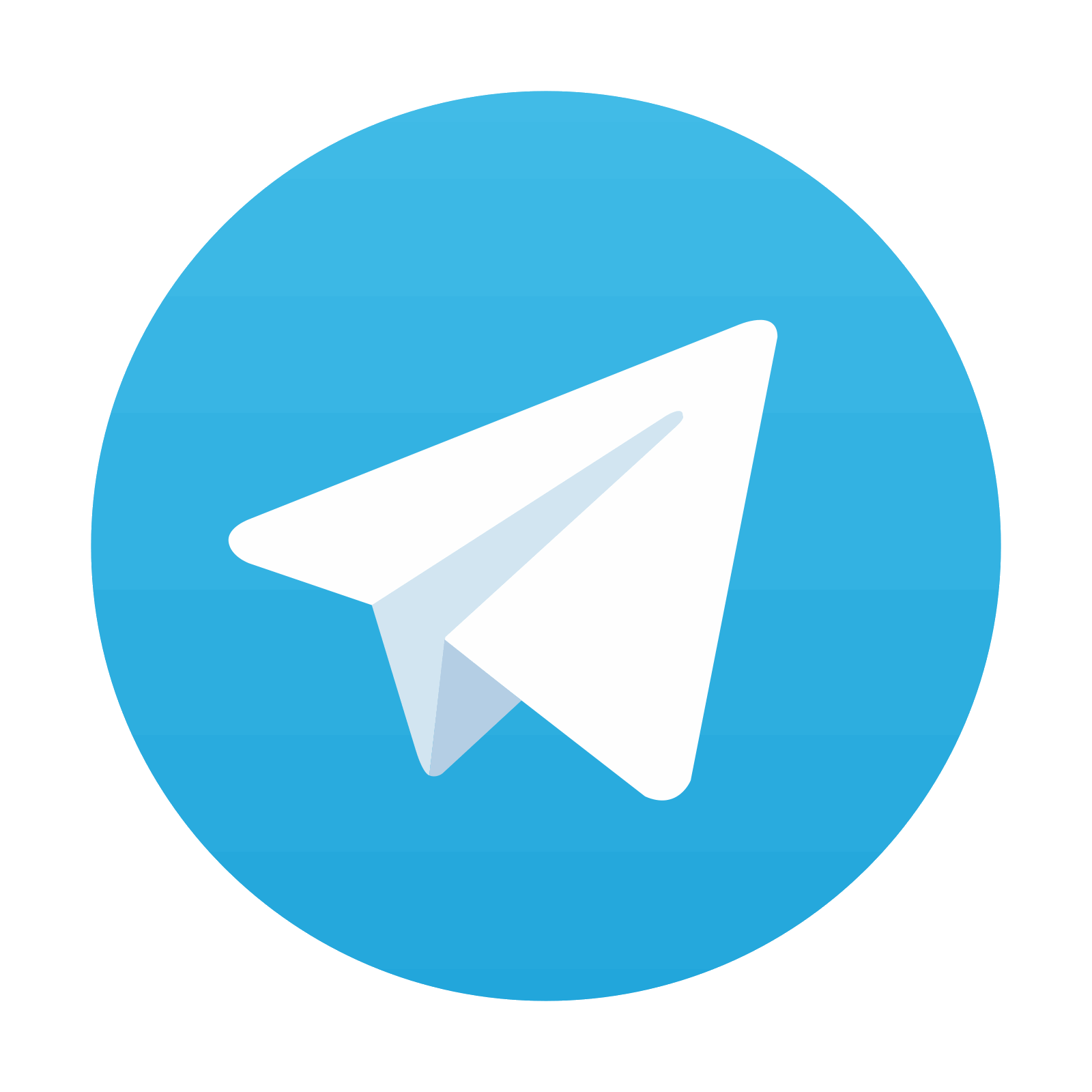
Stay updated, free articles. Join our Telegram channel
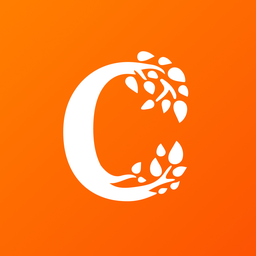
Full access? Get Clinical Tree
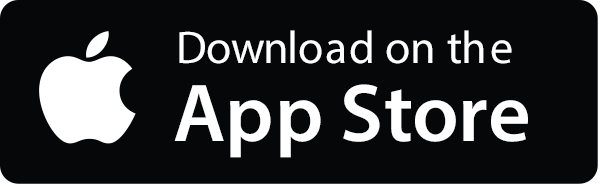
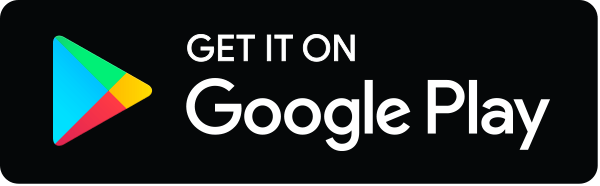
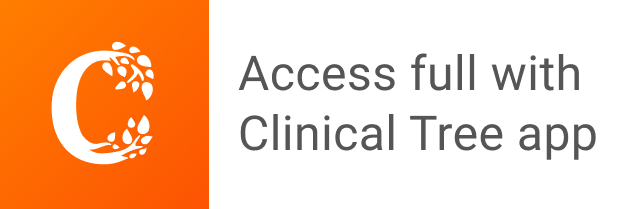