34 Future Growth in Navigated and Robotic Spinal Surgery
Abstract
As technology advances at a rapid pace, contemporary surgery is becoming more efficacious, precise, and safe, with new options offering promise for some of the most challenging diseases. In this chapter, the authors review several emerging technologies in navigated and robotic spinal surgery, discuss legal questions, and outline future challenges for an evolving field. Augmented reality, including modern heads-up displays such as Google Glass and Microsoft HoloLens, is first reviewed, along with recent systems able to superimpose 3D anatomy with the surgeon’s hand and instrumentation. Advances in imaging, including diffusion tensor imaging, are then explored, and potential utilities in tumor resection are discussed. Progress in nanotechnology is covered next, including nanoknife and nanoscaffolds, as well as nanorobots designed to selectively target tumor cells and administer a chemotherapy payload. Legal questions are then investigated, including Food and Drug Administration (FDA) approval and legal liability for robots. The chapter concludes with a discussion of ongoing challenges in robotic spine surgery, including cost-effectiveness, miniaturization, artificial intelligence, and ethical considerations of the robot as a surgeon.
34.1 Introduction
Augmented reality (AR) is the projection of computer-generated images onto the user’s view of the real world. Although only in its infancy, AR has been subject to growing interest in surgical training as well as certain procedures to improve precision and minimize trauma. 1 Several AR systems are being tested for spinal applications, including commercial heads-up displays (HUD) such as Google Glass and Microsoft HoloLens, as well as AR surgical navigation devices.
34.1.1 HUD AR—Google Glass and Microsoft HoloLens
Over the last several decades, the ergonomics of AR systems have evolved into wearable technology with increasing ease of use. Contemporary HUD devices are mounted over the eyes and allow the surgeon to view imaging overlays of CT or MR data without needing to look off the surgical field. 2 Google Glass (Google, Mountain View, CA) consists of a miniature computer, screen, and projector mounted onto a pair of glasses that allows the user to view projected images on the top right of their right eye (Fig. 34‑1). Google Glass has found utility in displaying intraoperative monitoring data for dorsal rhizotomy procedures, 3 as well as live, updating data from neuronavigation devices to monitor pedicle screw placement. 2

The Microsoft HoloLens (Microsoft, Seattle, WA) consists of a visor that can project three-dimensional (3D) images in space and has paired speakers to create virtual surround sound cues. The HoloLens utilizes gaze commands, such as head tracking, as well as gestures and voice inputs to allow the user to interact with projected images. The HoloLens has been used, for example, in a proof of concept study to project holograms of spinal deformity patients, allowing for surgical planning in a 3D space and later postoperative review. 4
34.1.2 Augmented Reality Surgical Navigation
A recently developed augmented reality surgical navigation system (Philips Healthcare, Best, the Netherlands) has been subject to recent study as an aid to pedicle screw placement. The system consists of multiple high-resolution video cameras mounted on a C-arm used to track markers placed on the skin around the surgical site. After creation of a 3D image with rotation of the C-arm, a 3D volume on the screen is seen, a screw insertion path is planned, and the C-arm automatically rotated to the axis of the planned screw. On the display screen, the screw path is then overlaid in real time over the patient’s underlying anatomy to guide screw trajectory and depth 5 (Fig. 34‑2). Initial results on a cadaveric model revealed superiority of the AR system in achieving optimal screw placement and avoiding breaches versus the freehand technique. 5

34.2 Diffusion Tensor Imaging
Diffusion tensor imaging (DTI) is a magnetic resonance imaging technique used to render 3D images of white matter tracts in the CNS. 6 The presence of a mass within a white matter tract causes deviations of the tract’s normal path through the brain or spinal column, allowing the surgeon to visualize the location of the tumor in 3D space based on altered tract pathways 7 (Fig. 34‑3). Visualization of white matter tracts helps the surgeon plan approaches to avoid injuring pathways which can create neurologic deficits.

While potentially useful as a surgical adjunct, DTI has been criticized as unreliable in its current state due to the noise involved in constructing 3D images. 9 , 10 Diffusion-weighted imaging on its own is low resolution, and when coupled with fiber orientation and complex tracking algorithms can produce results that are challenging to replicate. 10 For intradural masses, opening of the dura and release of cerebrospinal fluid (CSF) can also cause movement of the spinal cord, making images unreliable. These shortcomings have led some authors to argue that DTI is not refined enough to be used in routine clinical practice until acquisition and rendering techniques are improved. 9
Improvements in DTI under investigation include more robust models of data acquisition such as spatiotemporally encoded (SPEN) magnetic resonance imaging, which has been shown to provide more reliable data compared to DTI-rendered images. 11 SPEN improves image reliability by decreasing noise present in diffusion motion, resulting in more robust data acquisition in tissues that display chemical heterogeneity. 11 Other potential improvements include the addition of feedback mechanisms, such as ultrasound monitoring, which can account for spinal cord movement on dural opening and allow images to be updated with new positions.
34.3 Nanorobotics
Renowned physicist Richard Feynman, credited with introducing the concept of nanotechnology in 1959, suggested that machines might one day reduce in size to the extent that one could, in effect, “swallow the doctor.” 12 Once the subject of science fiction, nanotechnology has evolved from a futuristic concept into a mainstream research initiative with potential applications in all fields of science including medicine and surgery. Governments around the world are increasingly investing in nanotechnology, 13 , 14 while medical specialties are eagerly examining applications for the detection and treatment of disease. 13 , 15 , 16 , 17
Nanomaterials and devices are structured on the order of 10– 9 to 10– 7 meters (1–100 nanometers), 18 with a nanometer roughly the size of a molecule. 17 Due to their small size, nanoparticles and nanotools can decrease the risk of injury during surgery and make accessible anatomically challenging regions of the body. 19
Nanorobotics is the branch of technology dealing with the design, construction, operation, and application of robots that have components at or near the scale of a nanometer. To date, various nanomedicine applications have been investigated clinically and are being incorporated into nanorobotics for targeted drug delivery, imaging, diagnostics, tissue engineering, surgery, and intraoperative navigation. Polymeric nanoparticles, liposomes, and dendrimers can deliver drugs, growth factors, and genetic material across selective membranes including the blood–brain barrier. 15 , 20 For diagnosis, nanoparticles can be magnetized or conjugated to specific cell markers, and light-emitting quantum dots or metallic nanoparticles can be used as contrast agents or to detect specific biomolecules. 20 Nanoelectromechanical systems (NEMS) and microelectromechanical systems (MEMS), machines composed of miniaturized electrical and mechanical apparatuses such as actuators, beams, sensors, pumps, resonators, and motors, have been explored as tools to monitor physiological variables such as CSF pulsatility, intracranial pressure, weight load, and strain. 19 Finally, tools such as femtosecond laser systems, nanoneedles, and nanotweezers enable molecular ablation, dissection, penetration, and transfer at the subcellular level, and have been subject to growing interest. 18 , 20
34.3.1 Nanotechnology in Surgery
Nanotechnology is beginning to be used for spine surgery, including treatment for spinal cord injury, peripheral nerve repair, and neuronal regeneration. Platforms for individual axon repair have involved tools such as the 40-nm-diameter nanoknife and dielectrophoresis, which uses electrical fields to manipulate polarizable objects in space. 20 , 21 Fusion between ends of transected axons can be induced via electrofusion, polyethylene glycol, or lasers, among other methods. 21 In addition, nanoscaffolds consisting of carbon nanotubes, nanowires, or polymer nanofibers can provide a structural framework to promote axon regeneration or to augment bone tissue regeneration and enhance osseointegration of implants. 17 , 22
Biogenesis using nanomaterials can also be used in spinal fusions. One biomimetic approach involves nanocrystal-induced formation of organoapatite on surgically implantable structures such as foils and meshes. 16 , 20 , 22 Scaffolds formed by self-assembly of molecular nanofibers interspaced with mineralized hydroxyapatite crystals morphologically resemble collagen fibrils. 23 , 24 These scaffolds, when seeded with stem cells, have been demonstrated to generate cartilage and bone tissue in vivo, and therefore have found potential utility in improving bone growth after fusion, repairing annular defects, or regenerating the nucleus pulposus. 23 , 24
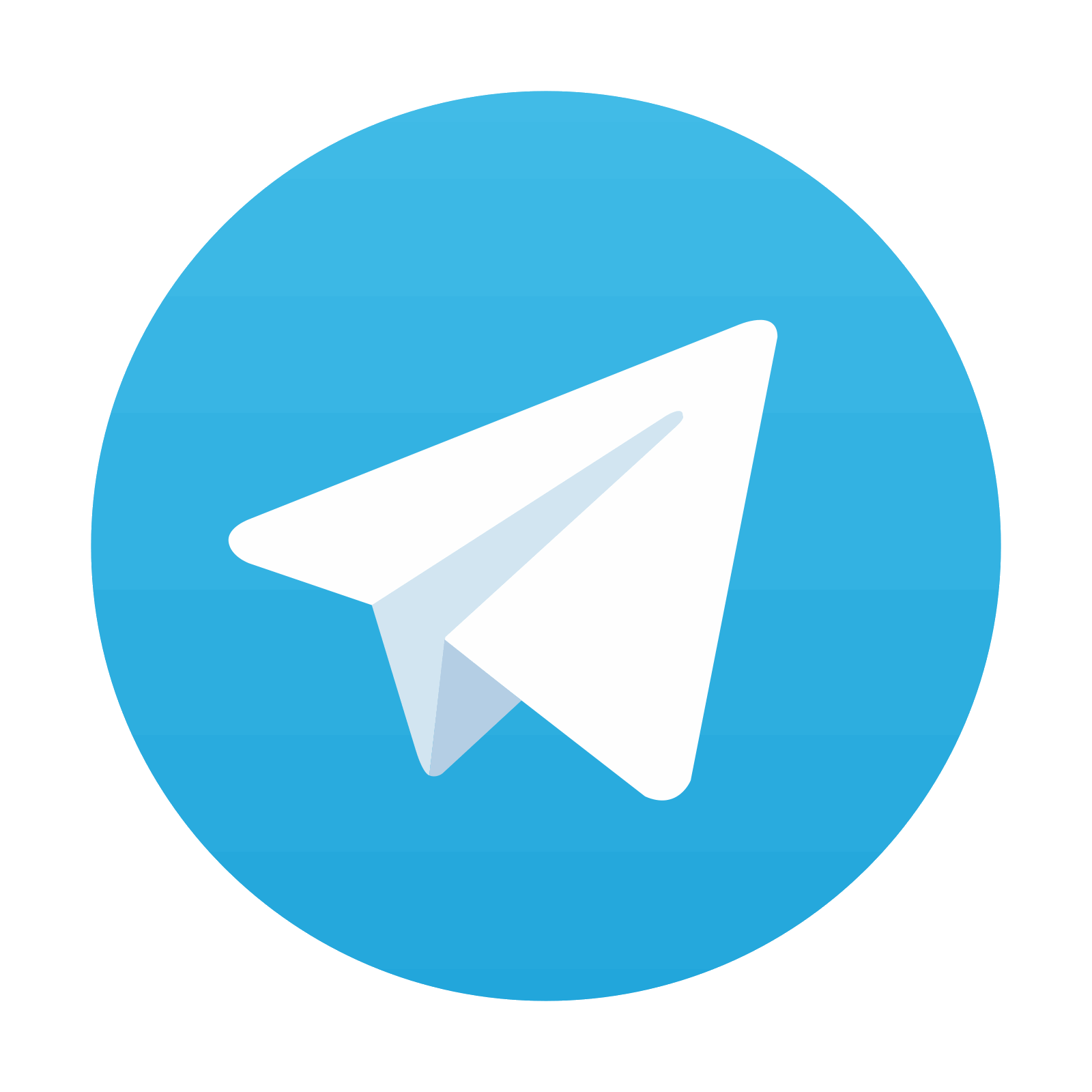
Stay updated, free articles. Join our Telegram channel
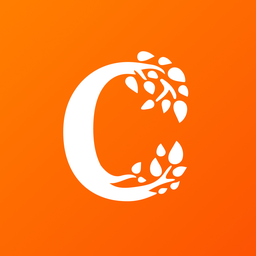
Full access? Get Clinical Tree
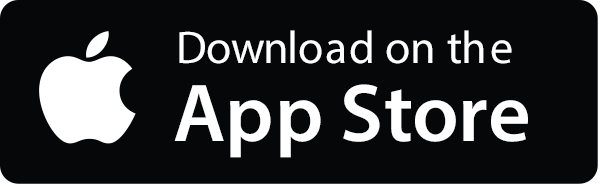
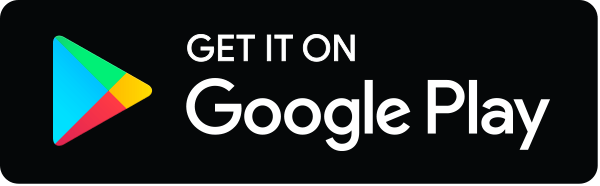
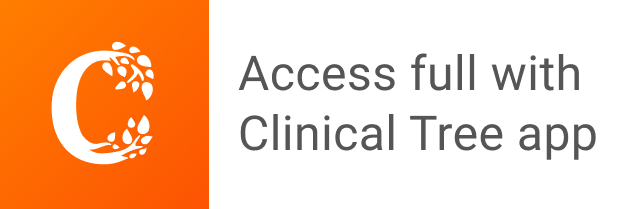