36 Intraoperative Magnetic Resonance Imaging–Guided Resection of Meningiomas
To improve is to change; to be perfect is to change often.— Sir Winston Churchill
The progress of neurosurgery has, to a large extent, depended on advances in technology, in particular, those related to lesion localization. Adapting these technologies to the operating room (OR) environment refined both lesion localization and resection control.1 In the surgical re-section of meningioma, intraoperative imaging provides an update to diagnostic images, optimizes craniotomy placement, and provides intraoperative evidence that surgical goals have been accomplished.
History
The first intraoperative magnetic resonance imaging (iMRI) system was installed at Brigham and Women’s Hospital, Boston, Massachusetts, in 1994.2 The system was based on a vertical biplanar (double donut) magnet, allowing the surgeon to operate within the 56 cm aperture, and thus integrated real-time imaging with the surgical procedure. Because surgery was conducted within the magnetic field, all surgical instrumentation and equipment, including the OR table, microscope, anesthesia equipment, and patient monitoring devices were required to be manufactured from MR-compatible material.3–5 Although the value of this technology was evident, it was not widely adopted, due, in part, to the restricted surgical space, the need for all instrumentation to be MR compatible, and the cost associated with installation and maintenance. General Electric (Milwaukee, WI), the manufacturer of this iMRI configuration, no longer markets the system.
Other low-field iMRI systems, based on magnets of variable field strengths ranging from 0.12T to 0.3T, were built or adapted for surgical use.6–8 These iMRI systems are of open configuration and have the magnet adjacent to or below the OR table. For example, the PoleStar iMRI system (Odin Medical Technologies, Yokneam, Israel) is based on a portable 0.12T magnet positioned below the OR table and surgical field.6 The very low field allows for use of standard neurosurgical instrumentation because magnetic pull does not affect tool manipulation. For imaging, the magnet is moved up into the surgical field. Although the system has many attractive features, the low magnetic field precludes advanced imaging sequences or images of diagnostic quality. Furthermore, magnet design makes imaging of the posterior fossa and upper cervical spine problematic.9
To obtain image resolution comparable to that of diagnostic MRI scanners, higher field 1.5T iMRI systems were developed.1,10,11 For example, the University of Calgary, in collaboration with the National Research Council of Canada, developed an iMRI system based on a movable, ceiling-mounted 1.5T magnet.1,11 The system included the design and manufacture of an MR-compatible OR table, radiofrequency (RF) coils specifically designed for OR use, and a custom-made gradient insert that increased the available working aperture. Together, these provided a patient-focused surgical environment with freedom to optimize patient positioning and improved signal-to-noise ratio.
The movement of the MR system obviated the need to transport the patient, thus enhancing patient safety, sterility, and efficiency within the OR. In addition, the configuration permitted the use of standard neurosurgical instruments and monitoring devices. The configuration, as adopted by other neurosurgical units, allowed sharing of technology between surgery and other disciplines. As a result, costs related to obtaining and maintaining the MR technology could be offset.
Recently, iMRI systems based on 3.0T magnets have been integrated into the OR.12–15 The 3.0T system at the University of Calgary is based on the same moving magnet configuration as that developed for 1.5T. The trend toward a higher magnetic field is based on the linear relationship between signal to noise and magnetic field strength.16 In general, improved signal to noise relates to improved image resolution and decreased acquisition time, and high performance gradients enhance diffusion tensor imaging,16 functional imaging,17 and multi-nuclear MR spectroscopy.18
The Magnetic Environment
Static Magnetic Field
The static magnetic field is the primary magnetic field of an MRI scanner. It is highest at the isocenter of the magnet and decays as distance from the isocenter increases in a nonlinear relationship.4 The area around the MRI scanner that is considered safe is outside the 5 gauss (5G) limit.3,4 A 3T magnet will have a larger restricted area (higher than 5G) compared with a 1.5T magnet. For 1.5T and 3T magnets, this restricted area has been considerably decreased through active magnetic shielding. Ferro-magnetic objects, including instruments and equipment, if brought within the 50G magnetic field, may become projectiles, accelerating toward the magnet.19 Based on this principle and to increase the margin of safety, ferro-magnetic instruments or equipment need to remain outside the 5G limit. In the case of the movable iMRI system, when the magnet is moved toward the patient to obtain images, noncompatible equipment and instruments are moved out of the new 5G limit that forms around the patient.
Gradient Magnetic Field
In addition to the static magnetic field, a variable gradient (according to a prescribed sequence) is applied in three directions (x,y,z). The frequency of variable gradient is close to that of audible acoustic frequency, which is responsible for the noise during image acquisition. The variable gradient also has the potential to induce voltage or current in tissues, creating heat and neural or muscular activation. It can also cause voltage changes in pacemakers, inadvertent instrument movement, and image artifact.3 To minimize the effect of the gradient magnetic field, U.S. Food and Drug Administration (FDA) guidelines for magnetic resonance equipment safety require all scanners to be programmed to function at a defined field.
Radiofrequency Electromagnetic Field
RF coils produce an electromagnetic field needed to excite hydrogen molecules within tissues, and the same coils, or different coils, are used to collect MR signal from the excited molecules as they relax. As relaxation rates differ between tissue types, soft tissue contrast is achieved.
In extreme cases, heat produced by an RF field can cause burns, interfere with electrical instruments (such as anesthetic equipment), and induce currents in looped conductors (such as electrocautery wires). Negative effects can be mitigated by avoiding looped wires and individually screening equipment for compatibility within the RF field.3
Anesthesia Considerations
The MR environment poses challenges for anesthetists as well as for the surgeons, making it important for them to be trained in device selection and limitations. These involve considerations related to the anesthesia machine, monitoring and infusion equipment, anesthetic agents, and patient positioning and exposure. Electric noise generated by pulsating electric and magnetic energy on conductors distorts the physiological waveforms on the monitors,20 in particular the electrocardiogram ST segment. Likewise, temperature control, blood pressure monitoring, tissue oxygenation, and ventilation can be affected in the presence of RF waves produced by an MR scanner. Accepted guidelines for patient safety, monitoring, and equipment have been published.3,20
The iMRI System at the University of Calgary
Central to the patient-focused iMRI environment at the University of Calgary was the design and manufacture of an integrated MR-compatible OR table ( Fig. 36.1A ). The table design, head fixation, and hydraulic table movement allow the patient to be positioned supine, lateral, and prone. The table is as wide as conventional OR tables, with a slim profile so as to optimize head placement within the magnet isocenter. Both three- and four-pin head fixation have been developed for use with the table and magnet. The provision of hydraulic actuators allows maximum positioning capability without backlash and the ability to smoothly reposition during microdissection. Thus the table becomes an important adjunct to microsurgery.
The iMRI system is based on a ceiling-mounted movable 3.0T magnet (IMRIS, Winnipeg, Canada), ( Fig. 36.1B ) capable of transfer into and out of the OR. The magnet measures 1.73 m in length and has an internal diameter of 90 cm. The working aperture is 70 cm with shielded gradients in place. The 5G restricted area measures 4 m × 3.5 m. The system provides a large homogeneous field of view measuring 50 × 50 × 50 cm at the magnet’s isocenter, and it includes high-performance 45 mT/m gradients.
RF coils allowing transmission and receipt of signal were developed and integrated into the head fixation apparatus. The RF-coil design ( Fig. 36.1C ) includes a transmit body coil within the bore of the magnet and several receiving coils. These receiving coils (IMRIS, Winnipeg, Canada) are able to be separated into two components with four channels each, such that the bottom coil is left in place during surgery. Increased number of signal channels, improved software design, and parallel imaging techniques permit high-resolution imaging with decreased total acquisition time.

The OR is outfitted with whole-room copper shielding (Gaven Industries, Saxonburg, PA), making use of wave guides, filters, and the conversion of electrical signals to fiber optics to accommodate necessary electrical devices. The iMRI workstation is located adjacent to the operating room, separated by a transparent copper mesh-impregnated glass panel. Wooden doors ( Fig. 36.1D ) separate the magnet alcove from the operating room for safety.
Imaging sequences are provided by Siemens 3.0T software (Syngo MR B15V, Siemens, Erlangen, Germany; optimized for iMRI application by IMRIS, Winnipeg, Canada). Intraoperative imaging utilizes standard and advanced imaging sequences, including T1-weighted, T2-weighted, fluid-attenuated inversion recovery (FLAIR), time-of-flight MR angiography, diffusion-weighted images, and diffusion tensor images (DTI). Postprocessing software permits the rapid generation of three-dimensional tractography ( Fig. 36.2 ). Gadolinium can be used to define unsuspected residual tumor at various stages of surgical dissection. However, if administered during the planning stage before surgery, it may diffuse into normal tissue, making the identification of residual tumor difficult in intradissection scans.
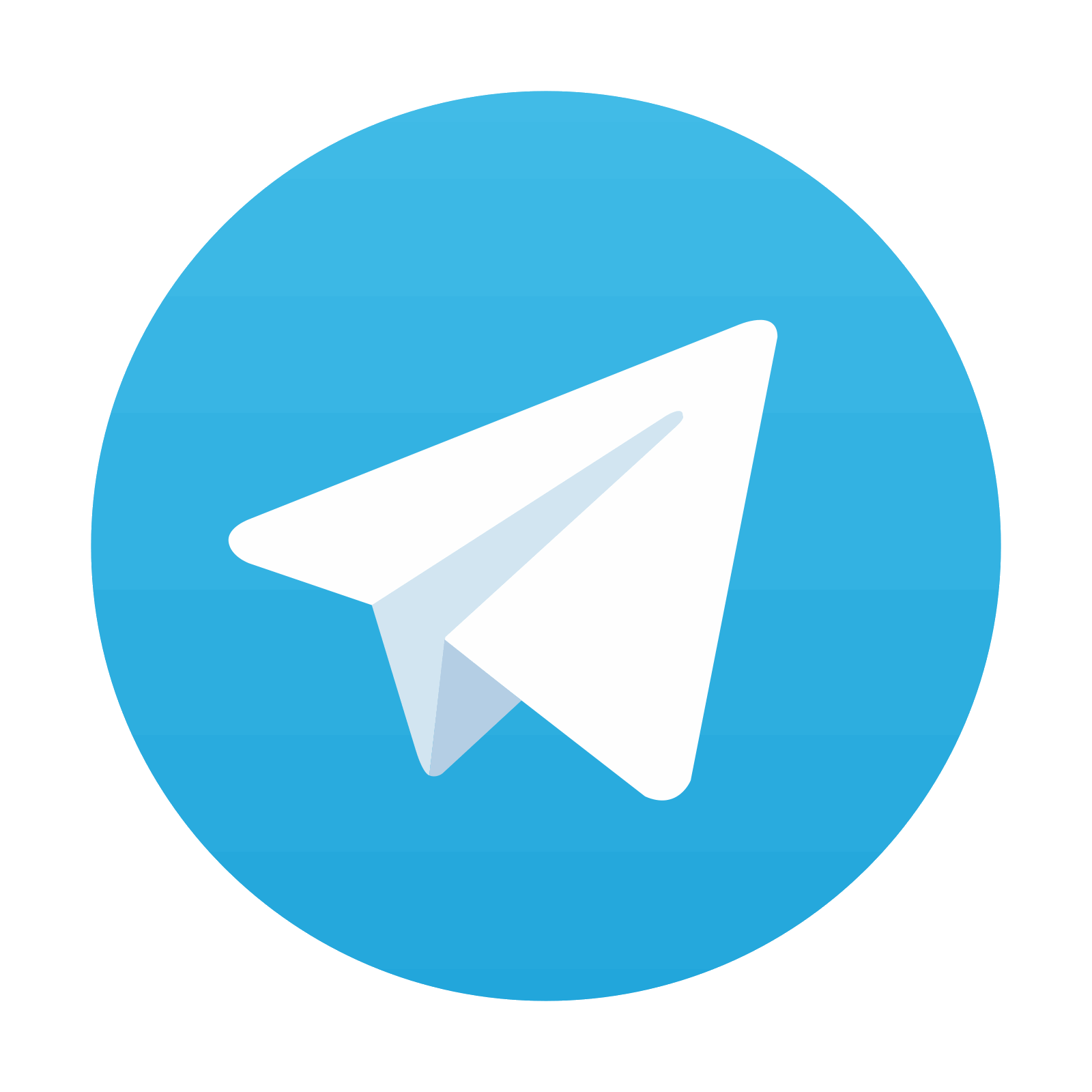
Stay updated, free articles. Join our Telegram channel
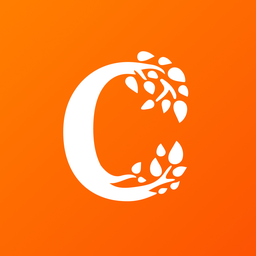
Full access? Get Clinical Tree
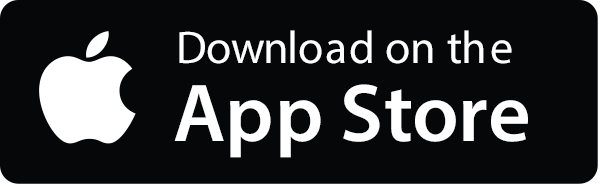
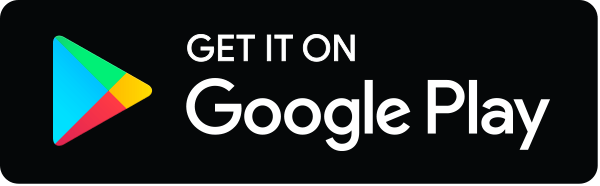
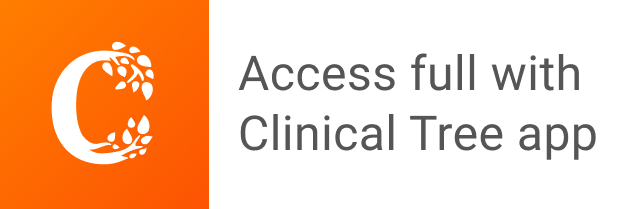