4 Genetics of hydrocephalus
Adult Hydrocephalus, ed. Daniele Rigamonti. Published by Cambridge University Press. © Cambridge University Press 2014.
Introduction
Hydrocephalus is a common human neurological abnormality that requires medical intervention, yet remains an elusive pathological condition which scientists and physicians aim to understand. Improvements in both diagnostic techniques and surgical treatments of hydrocephalus have led to better long-term outcomes and increased prevalence of patients harboring this disease, however our knowledge of hydrocephalus is still very limited [1,2]. Human hydrocephalus can be classified as syndromic versus nonsyndromic, and congenital versus acquired [3]. Syndromic hydrocephalus is usually associated with other major developmental and structural abnormalities such as intraventricular hemorrhage, congenital neoplasia, Chiari malformation, Dandy–Walker malformation, prenatal and perinatal infections, and other severe developmental syndromes. This chapter focuses on nonsyndromic or uncomplicated hydrocephalus, which can be further subclassified into congenital or acquired. Congenital hydrocephalus is defined as being present at birth with or without associated anomalies while acquired hydrocephalus is defined as cases that occur after normal development of the brain and ventricles.
Hydrocephalus is a heterogeneous disease with multiple possible causes [4,5]. A growing body of evidence suggests that the pathogenesis of hydrocephalus is a complex interaction of genetic and environmental factors. Known environmental factors such as maternal alcohol abuse [6], X-ray radiation [7,8], infection [9], nutritional deficiencies [10], and side effects of antibiotic and antineoplastic drugs [11,12] have all been linked to hydrocephalus. It is estimated that about 40% of hydrocephalus cases may have a significant genetic component [13]; we previously have described at least 43 mutants/loci linked to hereditary hydrocephalus in animal models and humans [14]. Among these 43 loci, 10 causative genes in the pathogenesis of hydrocephalus have been defined, 9 of which have been identified in animal models [14]. In humans, only the L1CAM gene localized to Xq28 encoding L1 protein and comprising approximately 5–15% of congenital hydrocephalus cases has been positively associated with this condition (X-linked, HSAS1, OMIM) [15–18].
Several reports in human kindreds harboring an autosomal recessive, autosomal dominant, additional X-linked, and mitochondrial patterns of inheritance have been reported; however, the genes and loci responsible for these forms of transmission have not been clearly identified [19–27]. Differential expressivity in gene or gene products has also been implicated as a causative factor.
Genetic epidemiology
Although significant progress has been made in our understanding of molecular pathophysiology of hydrocephalus, the genetic study of hydrocephalus has been hindered by a lack of sufficient epidemiological evidence. The genetic etiology for the majority of hydrocephalus cases remains elusive.
Twin studies
Comparative twin studies have been performed to analyze the genetic influences in congenital structural defects including hydrocephalus [28,29]. Familial inheritance of hydrocephalus was first suggested almost sixty years ago by comparing the concordance in collective pairs of monozygotic twins [30,31]; however, the presence of hydrocephalus was later challenged in some of these twins [32]. In a follow-up study, after eliminating twin pairs in which the hydrocephalus was found to be secondary to some other etiological factor, congenital hydrocephalus in seven pairs of monozygotic twins was found, supporting the notion that genetic factors may play a major role in some cases of congenital hydrocephalus [32]. Recently in a single-institution 3-year retrospective study, a possible concordance for congenital hydrocephalus was observed among three twin pairs, one monozygotic twin pair both with hydrocephalus and two dizygotic twin pairs in each of which only one had hydrocephalus, suggesting a potential genetic influence in hydrocephalus [33]. Moreover, another report showing that a dizygotic twin pair, each with hydrocephalus, were homozygous for the FAC gene mutation, which provides molecular proof that VACTERL with hydrocephalus may be the result of severe Fanconi anemia [34]. This report also raises the possibility that dizygotic twin pairs, each with hydrocephalus, may be caused by genetic concordance due to homogeneity in a certain genomic region.
Epidemiology
It has been suggested that the majority of congenital hydrocephalus (CHC) is caused by some kind of a genetic defect [35]. A study of 74 patients with uncomplicated CHC found that there was a significant increase (five times the population frequency) of CHC among first cousins. Once X-linked inheritance has been excluded in uncomplicated CHC, the overall empirical risk of recurrence is approximately 1 in 50 [36]. In a prospective study, results suggest that couples who have had one child with hydrocephalus have a recurrence risk of 4% [5]. Our group performed a 10-year retrospective study (1997–2007) in Mississippi with a total of 4312 hydrocephalic patients, 710 with congenital hydrocephalus. Among those with CHC, we identified 47 congenital hydrocephalus patients with siblings or first to third degree relatives who also have hydrocephalus, which we further subcategorized into familial cases. Of these familial hydrocephalus cases, we found 14 siblings including 6 sets of twins and 1 set of triplets, 9 first cousins, 22 second cousins, and 6 third cousins. Four of these families had three affected members with hydrocephalus. The pedigrees of each of these 47 families were developed, with the largest cohort consisting of 29 members. However, only two families showed an X-linked inheritance pattern, indicating that most of them were pedigrees with inheritance patterns other than X-linked [2].
One recent study examined familial aggregation of primary CHC in an unselected, nationwide population in Denmark from the Danish Central Person Register. This study identified all children born in Denmark between 1978 and 2008 and their family members (up to third degree relatives). Recurrence risk ratios (RRRs) of congenital hydrocephalus were estimated and a log-linear regression model was used to quantify the genetic effect and the maternal effect. The data showed that among nearly two million live births, 2194 had a diagnosis of idiopathic congenital hydrocephalus (1.1%). Of those, 75 (3.4%) had at least one other family member with CHC. Significantly increased RRRs of CHC were observed for same-sex twins, and for first and second degree relatives. A maternal component was supported by the facts that RRRs for opposite-sex twins (37.3, 95% CI 11.9–116.7) were significantly higher than for other first degree relatives, and that RRRs for maternal half-siblings (8.4, 95% CI 3.7–18.7) were significantly higher than for paternal half-siblings (3.0, 95% CI 0.8–12.2). This is the first large-scale genetic epidemiology study in an unselected, nationwide population. Taking advantage of extensive and detailed records from the national database in a relatively homogeneous population, this study provided the strongest evidence so far of familial aggregation of CHC, supporting the existence of a genetic component to the etiology. In addition, the pattern of association suggests a strong maternal component contributing to the familial aggregation [37].
Genetics in hydrocephalus
Human hydrocephalus
Familial hydrocephalus has long been suggested as a heritable disease [38,39], with heterogeneous causes [40,41], which may result from distinct monogenic or multifactorial disorders [14]. However, limited knowledge exists about the genetic and molecular mechanisms that may cause human hydrocephalus. Identification of large numbers of heritable forms of hydrocephalus would pave the way for elucidation of cellular functions of gene products that lead to alteration in cerebrospinal fluid (CSF) dynamics. Clarification of perturbed cellular pathways may lead clinicians to improved genetic counseling for patients, prenatal diagnosis, and in developing potential novel therapeutic approaches for hydrocephalus.
Idiopathic normal pressure hydrocephalus
Acquired hydrocephalus is also termed adult-onset or idiopathic normal pressure hydrocephalus (iNPH). In contrast to CHC, familial cases of iNPH are not well identified and the genetic basis is totally unknown [42]; only a few familial iNPH cases have been reported to date [26,43–45]. We first reported a large five-generation kindred with essential tremor (ET) presenting during the teen years and the subsequent appearance of iNPH when elderly (>65 years), in an autosomal dominant fashion. Through clinical and genetic analysis, we defined this kindred as a new essential tremor–idiopathic normal pressure hydrocephalus (ETINPH) disorder [43]. We then performed genome-wide linkage scans using two different genotyping microarrays and two- or multipoint linkage analysis strategies and mapped the ETINPH locus to chromosome 19q12–13.31. Fine-mapping in this region revealed a maximum two-point LOD score of 2.8 at rs2023865 and a maximum multipoint LOD score of 17.9 at rs9304878. By haplotype construction, an approximately 17-cM critical region was defined for ETINPH locus. This region contains several neuronal genes that constitute tantalizing etiological candidates for ETINPH [44]. Currently we have deep-sequenced this entire interval with next generation sequencing technology and are analyzing data to identify the genetic variant that causes this ETINPH phenotype. Another large family consisting of four patients with adult-onset iNPH in three generations has been reported recently, supporting the existence of familial iNPH and the genetic components of iNPH [45]. Interestingly, a recent report using a candidate gene approach identified a small familial case of two sisters with iNPH who were homozygous for the apolipoprotein E (ApoE) e3 allele on chromosome 19 [46]. Similarly, another recent study with 15 iNPH patients found that iNPH is associated with the homozygous ApoE 3/3 genotype [47].
Another report has suggested a genetic association between iNPH and the ApoE e4 allele [48]. Building on previous finding that homozygosity of the A allele of the alpha-1-antichymotrypsin (ACT) gene and of allele 1 of the presenilin-1 (PS-1) gene are associated with an increased risk for late-onset Alzheimer’s disease (AD), the distribution of ApoE, ACT, and PS-1 genotypes and the corresponding allele frequencies in 13 iNPH patients were analyzed. Only an increased ApoE e4 allele frequency was observed in iNPH patients versus controls, thus suggesting that the ApoE e4 allele may also be involved in the pathogenesis of iNPH [49].
Congenital hydrocephalus
Congenital hydrocephalus (CHC) is far more common than iNPH and its incidence has been estimated to be 3–5 cases per 1000 live births [50]. CHC is usually the consequence of deficient brain development and perturbed cellular function, implicating the important roles that CHC genes play during brain development. Since there is heterogeneity among clinical phenotypes, more genetic loci are expected in CHC. In general, the recurrence risk for CHC excluding X-linked hydrocephalus is low. Empiric risk rates range from <1% to 4% [51–53], demonstrating the rarity of heritable congenital hydrocephalus [13,17,20,21]. However, multiple human kindreds with CHC have been reported [13,17,19–27,38,54–67]. One kindred was reported in which congenital hydrocephalus was transmitted in an autosomal dominant fashion. This condition was associated with aqueductal stenosis but was not associated with mental retardation or pyramidal tract dysfunction. The lack of mental retardation and pyramidal tract dysfunction was in contrast to X-linked or recessive CHC with stenosis of the aqueduct of Sylvius where these abnormalities are commonly seen [60]. Another study identified a kindred with a microdeletion of 8q12.2–q21.2 that subsequently developed hydrocephalus. This trait was also transmitted in an autosomal dominant fashion [68]. One genetic mutation associated with human CHC has been identified so far, that is for X-linked human congenital hydrocephalus. Molecular genetic studies have revealed that the responsible gene for this X-linked human congenital hydrocephalus is L1CAM (L1 protein) at Xq28 [69]. The mutations are distributed over the functional protein domains. The exact mechanisms by which these mutations cause a loss of L1 protein function are still under investigation. In addition to the L1CAM gene at Xq28, there is another form of X-linked hydrocephalus linked to the proximal region at Xq27.3 [70]. Interestingly, in another three-generation pedigree of hydrocephalus (with megacisterna magna [MCM]) with autosomal or X-linked dominant inheritance, L1CAM and FOXC1 gene involvement in the pathogenesis of the disease was excluded, suggesting a different locus for CHC [62]. Uncomplicated CHC due to obstruction of the third ventricle has been found in two separate familial cases, one of which presented as an autosomal recessive trait [20,59]. Current known loci of hydrocephalus in humans are summarized in Table 4.1.
Table 4.1 Summary of current known loci of hydrocephalus in humans
a Clinical form: C, congenital; AO, adult-onset.
b Genetic trait: AR, autosomal recessive; AD, autosomal dominant; MIT, mitochondria.
Animal hydrocephalic models
To date, the majority of identified hydrocephalus loci and genes are from genetic analysis in hydrocephalic animal models. Relative easy access and manipulation in animal models has led to the identification of large numbers of heritable forms of hydrocephalus.
Quantitative trait locus (QTL)
The hydrocephalic Texas strain (HTX) rat model of inherited congenital hydrocephalus demonstrates a complex mode of inheritance and associated ventricular dilatation with abnormalities in the subcommissural organ. The severity of hydrocephalus in the HTX rat seems to be influenced by different genetic loci [71–73]. One study suggested that the HTX strain is a homozygous carrier of an autosomal recessive hydrocephalus gene with incomplete penetrance [74]. In another study, through backcrossing the HTX rat with the non-hydrocephalic Fischer F344 strain, with quantitative trait locus (QTL) mapping, potential CHC loci were identified on chromosome (Chr) 9 (peak markers D9Rat2), 10 (between markers D10Rat136 and D10Rat135), 11 (peak markers D11Arb2 and D11Rat46), and 17 (peak markers D17Mit4 and D17Rat154).
The genetics of hydrocephalus in an inbred strain of Wistar-Lewis rats (LEW/Jms) suggests genetic traits as an autosomal recessive [75] or semidominant or multigenic (possible QTL) trait with a possible locus on sex chromosomes [76], but none of the loci have been localized. In inbred mouse models by back-crossing strains (A/J and C57BL/6J), three QTL loci associated with congenital hydrocephalus have been identified and labeled as Vent8a, Vent4b, and Vent7c. As a major QTL controlling variance in ventricular size, Vent8a is located on Chr 8 (near the markers D8Mit94 and D8Mit189). The other two loci, Vent4b and Vent7c, show strong epistatic interactions affecting ventricular size in the developing embryo. Vent4b is located on Chr 4 (near D4Mit237 and D4Mit214), and Vent7c is located on Chr 7 (between D7Mit178 and D7Mit191) [77].
Monogenic congenital hydrocephalus
The autosomal recessive congenital hydrocephalus-1 (hy1) mouse has a dome-shaped head seen at birth or during the first 2 weeks due to dilatation of the entire ventricular system [78,79]. A more severe phenotypic form, the hydrocephalus-2 (hy2) mouse [80,81], and an obstructive hydrocephalus (oh) mouse with communicating hydrocephalus and secondary aqueductal stenosis have also been described [82,83]. Genetic loci on these non-inbred mouse strains have not been identified yet.
The transgenic mouse line OVE459 is generated by a Bdnf transgene-induced insertional mutation on a single locus on mouse chromosome 8 (near marker D8Mit152), and suggests an autosomal recessive trait in congenital hydrocephalus. The OVE459 insertion locus overlapped with that of autosomal recessive hydrocephalus-3 (hy3) mouse and phenotypically shows lethal communicating hydrocephalus with perinatal onset [84,85]. This transgene insertion resulted in a rearrangement of Hydin exons in OVE459 mice. Subsequently, a single CG base-pair deletion in exon 15 of Hydin was also identified in hy3 mice, implicating Hydin as hy3 gene [86,87].
An autosomal recessive congenital hydrocephalus (ch) mouse strain was identified many decades ago [84,85,88]. The mutation on winged helix/forkhead transcription factor gene, Foxc1 (Mf1), on chromosome 13 has been identified in ch mouse [89,90]. This finding is supported by another report of six children with hydrocephalus from three different families that have sub-telomeric deletions from chromosome 6p. Three forkhead genes within this region (FOXF1 and FOXQ1) or proximal to it (FOXC1) were evaluated as potential candidate disease genes but no disease causing mutations were identified [91]. A recent report indicates that one of the three forkhead genes, FOXC1, is required for normal cerebellar development. FOXC1 mutations have been shown to contribute to chromosome 6p sub-telomere deletion hydrocephalic phenotype, such as Dandy–Walker malformation [92].
The autosomal recessive hydrocephalus with hop gait (hyh) mouse exhibits dramatic dilation of the ventricles at birth and invariably develops a hopping gait. The hyh mouse shows a markedly small cerebral cortex at birth, which indicates altered development of the neuronal cells, and dies postnatally from progressive enlargement of the ventricular system. It has been hypothesized that the small cortex phenotype is the result of developmental events in which neural progenitor cells withdraw prematurely from the cell cycle, producing more early-born, deep-layer cerebral cortical neurons but depleting the cortical progenitor pool. Genetic linkage analysis localized the hyh locus on mouse Chr 7, and subsequently the hyh gene was identified as α-Snap (soluble NSF attachment protein α). α-Snap protein is essential for apical protein localization and cell fate determination in neuroepithelial cells [93]. Homozygous mutant mice harbor a missense mutation, M105I, in a conserved residue in one of the α-helical domains. The hyh mutant is not a null allele and is expressed; however, the mutant protein is 40% less abundant in hyh mice, suggesting a possible haploinsufficiency mechanism [94].
The autosomal recessive hemorrhagic hydrocephalus (hhy) homozygous mutant mouse has dilated lateral ventricles and a patent aqueduct, with no histological abnormalities either in the subarachnoid space or in the choroid plexus. Multiple hemorrhages in the meninges and throughout the brain parenchyma have also been observed in the advanced stages of hydrocephalus. The hhy locus has been localized on mouse Chr 12 [95], and hhy gene has been identified as Ccdc85c encoding a protein at apical junctions of radial glia [96]. Several loci on chromosomes 4, 5, 7, 11, 15, and 17 of mouse MSM strain were recently identified as having a significantly higher incidence of hydrocephalus, by using a congenic technique of backcrossing MSM with the C57BL/6J (B6) strain [97]. Current known loci of hydrocephalus in animal models are outlined in Table 4.2.
Table 4.2 Summary of current known loci (or mutants) of hydrocephalus in animal models
AR, autosomal recessive; AD, autosomal dominant; QTL, quantitative trait locus.
Genetic basis for pathophysiology of hydrocephalus
The neuropathology of hydrocephalus has been extensively explored. Cerebral ventricle dilatation secondary to disturbed CSF flow has been observed as an inheritable trait in a variety of laboratory animals (as well as in humans) as mentioned previously. In most cases, defective development of the cerebral aqueduct of the subarachnoid space has been observed [98]. Affected individuals have severe developmental delay and may have radiographic findings of hydrocephalus [40]. The pathophysiology of hydrocephalus in the ventricular system has been extensively studied through either down- or up-regulation of certain targeted gene expression, followed by comparative morphological and molecular studies.
Loss of the key molecular cues during early development
In all forms of hydrocephalus, the majority of phenotypic abnormalities are caused by a loss of function of certain genetic components. Below we discuss the effects of those deficient molecular cues on the initial pathogenic tissue/organs that eventually lead to hydrocephalus.
Subcommissural organ (SCO) defects
The subcommissural organ (SCO), a small gland in the diencephalic plate inferior to the pineal organ, is located on the ventral side of the posterior commissure and affects the opening of the cerebral (sylvian) aqueduct. The SCO facilitates the patency of the sylvian aqueduct and normal CSF circulation/metabolism through its secretory activity [99]. Stenosis of the sylvian aqueduct is a common form of CHC in humans. Some mouse models of CHC indicate that aqueduct stenosis is associated with abnormal development of the SCO [100]. The HTX rat shows a reduction in the secretory cells of the SCO. Comparative analysis on serial brain sections through aqueduct regions containing the SCO from HTX rats and normal Fischer F344 rats has found that reduced SCO glycoprotein immunoreactivity precedes both aqueduct closure and expansion of the lateral ventricles in the HTX rat [101,102]. The LEW/Jms and HTX mutant rat models were identical with regard to the form of presentation and progression of hydrocephalus in the postnatal period; the pathogenesis of these two conditions in the fetal period was different. The LEW/Jms rats showed primary congenital aqueductal stenosis in early prenatal life and the hydrocephalic state appeared before pulmonary maturation was completed [76,103,104]. Several cases of autosomal recessive or autosomal dominant CHC due to aqueductal stenosis have been reported in humans [13,19,27,60,63], although the genes causing this type of hydrocephalus are currently still unknown.
Huntingtin (htt) protein, which causes Huntington’s disease (HD) primarily by a gain-of-function trinucleotide repeat expansion, plays a major role during embryogenesis through its interaction with multiple cellular partners. Conditional removal of mouse htt protein in Wnt1 cell lineages using the Cre-loxP system results in congenital hydrocephalus associated with abnormalities in the choroid plexus (CP) and SCO and an increase in CSF production by the CP [105]. The cyclin-dependent kinase inhibitor (CKI) p57 regulates the activities of E2F1 and p53 to ensure the proper proliferation and survival of cells in the SCO. Conditional disruption of p57 gene in the brain results in severe nonobstructive hydrocephalus [106]. Likewise, presenilin-1 (PS1), which is responsible for the development of early-onset familial Alzheimer’s disease, regulates metabolism of several important brain molecules during neurogenesis, including APP, Notch, cadherins, and β-catenin. Mice conditionally lacking PS1 in Wnt1 cell lineages with the Cre–loxP system also showed congenital hydrocephalus and SCO abnormalities [107]. Pax6, a developmentally regulated transcription factor, is expressed in secretory cells of the SCO. Mice functionally lacking Pax6 protein (homozygous knockout) failed to develop the SCO. Mice with decreased Pax6 protein level (heterozygous knockout) have defective development of the SCO, indicating that development of the SCO requires normal Pax6 gene-dosage [108]. Regulatory factor X3 (RFX3), a winged helix transcription factor, is expressed in the ciliated ependymal cells of the SCO and CP. Rfx3-deficient mice suffer from congenital hydrocephalus of variable severity resulting from ependymal cell defects of the SCO and CP perturbing CSF production or circulation [109]. The CYP2J2 transgene interferes with the expression of a brain-specific isoform of the regulatory factor X4 (RFX4). This brain-specific isoform is called variant transcript 3 or RFX4_v3 and is crucial for normal brain development as well as for the genesis of the SCO. Loss of a single allele prevents formation of the SCO and leads to congenital hydrocephalus in an autosomal dominant fashion. This obstructive hydrocephalus appears to be secondary to failure of development of the SCO [110]. Msx1, a homeobox gene that is strongly expressed in the SCO and CP [111,112], controls a genetic hierarchy involving bone-morphogenetic-protein (BMP) and sonic hedgehog (Shh) signals [113]. Msx1-deficient mice showed absence or malformation of the SCO and of the posterior commissure (PC), collapse of the cerebral aqueduct, and development of hydrocephalus [114,115]. Msi1 (Musashi1), a member of the Musashi family of RNA-binding proteins, is strongly expressed in neural precursor cells. Homozygous Msi1 mutant mice developed obstructive hydrocephalus with aberrant proliferation of ependymal cells surrounding the sylvian aqueduct [116]. Dlg5, a conserved coiled-coil MAGUK protein, binds to syntaxin 4, a t-SNARE protein that regulates fusion of transport vesicles. Therefore, Dlg5 may facilitate interaction with the t-SNARE transport vesicle fusion machinery. Dlg5-deficient mice had closure of the aqueduct, accompanied by loss of ependymal cells and cilia, leading to severe hydrocephalus with hopping gait, similar to hydrocephalic hyh mice [117].
Ciliopathies
Cilia are complex cellular organelles protruding from the apical surface of cells with the polarized microtubule backbone (axoneme), through intraflagellar transport (IFT). Cilia play major roles in cell locomotion and fluid movement. Defects in cilia, or ciliopathies that are caused mainly by mutations in IFT genes [118], are associated with a range of human diseases, including hydrocephalus [119–121]. The hydrocephalus can be caused by the malfunction of cilia in ependymal cells [122–125]. Within the neonatal brain, ependymal cells form the epithelial lining of cerebral ventricles and cilia move coordinately on the apical surface to facilitate circulation of the CSF. The mouse hydrocephalus-3 (hy3) protein, hydin, is confined to the ciliated ependymal cell layer lining the lateral, third, and fourth ventricles. Hydin is a large protein that localizes in cilia/flagella and is not closely related to any previously known protein. Interestingly, a 314 amino acid domain identified within hydin shows a strong homology to caldesmon, an actin-binding protein [126], suggesting its potential interaction with the cytoskeleton [86,87]. The hydin-associated projection may play an important role in regulating switching of dynein activity [126]. Cilia are impaired in motility and incapable of generating fluid flow in hydin mutants [127,128]. Likewise, mutations in sperm-associated antigen 6 (Spag6) [129] also show lack of ependymal cell cilia, resulting in hydrocephalus. PolL (DNA polymerase λ) plays an essential role in the maintenance of genome integrity during development. Homologous PolL mutant mice developed hydrocephalus due to defective inner dynein arms of cilia from the ependymal cell layer [130].
The protein of axonemal heavy chain 5 gene (Mdnah5) as the hydrocephalus inducing gene, dynein, is also specifically expressed in ependymal cells. Mdnah5 is essential for ultrastructural and functional integrity of ependymal cilia. In Mdnah5 mutant mice, lack of ependymal flow causes closure of the aqueduct resulting in a defective ependymal flow and hydrocephalus [131]. The higher incidence of aqueduct stenosis and hydrocephalus formation in patients with ciliary defects proves the relevance of this mechanism in humans [131]. Pcdp1 (primary ciliary dyskinesia protein 1) protein localizes the cilia of brain ependymal cells and plays an important role in ciliary and flagellar biogenesis and motility. Loss of Pcdp1 results from ciliary dysfunction and leads to hydrocephalus [132].
Hedgehog signaling. Hedgehog (Hh) signaling requires the IFT machinery to shuttle GLI proteins and SUFU to the ciliary tip. Mutations in Hh signaling, anterograde and retrograde IFT motors (such as Kif3a [kinesin family member-3A], Dync2h1 [dynein cytoplasmic-2 heavy chain-1], ptch1 (Hh receptor patched-1]) would result defective cilia [133]. It was found that deficiency of ptch1 leads to defective cilia function in ependymal cells, resulting in hydrocephalus, through crossing quaking viable mouse (qkv/v) with ptch1+/− mice [134]. However, the qkv mouse contains a deletion of ~1.1 Mb in the proximal region of chromosome 17, affecting the expression of three genes: quaking (Qk), Parkin-coregulated gene (Pacrg) and parkin (Park2). One experiment showed that transgenic expression of Pacrg was necessary and sufficient to rescue the CHC phenotype in the qkv mutant, implicating the role of Pacrg in the pathogenesis of hydrocephalus [135]. Pacrg protein in the mouse has been localized to the motile ependymal cilia lining the ventricles of the brain as a component of the flagella axoneme. Loss of Pacrg in defective cilia is associated with impaired ciliary mediated CSF flow and the development of hydrocephalus [135]. Sonic hedgehog (Shh) signaling correlates spatially and temporally with fissure formation through its activators, GLI2 and GLI3, and its receptor, smoothened (SMO). Conditional removal of Gli2 [136] and Gli3 [137] leads to hydrophilic phenotypes; furthermore, hydrocephalus is one of the clinical diagnostics of patients with GLI3 mutation [138]. Mtf2 plays essential roles during early embryogenesis through its genetic interaction with Shh signaling; Mtf2 mutant mice exhibit several neurological defects including hydrocephalus [139].
Wnt signaling. Wnt signaling not only regulates neurogenesis itself, but also coordinates with sonic hedgehog (Shh) signaling to regulate the development of the brain [140,141]. Deletion of Wnt1 in mice revealed severe abnormalities in the development of brain, including hydrocephalus-like phenotype [142]. Abnormal cilia and impaired CSF movement in the brain are also observed in mutations in Ift88 gene (Tg737 or Polaris mutant mouse), due to altered function of the CP epithelium, as evidenced by hydrocephalus [118, 121,143]. The winged helix family of transcription factors has also been found to play an important role in ciliary development in CP. Hydrocephalic phenotype was observed in the forkhead homologue (hfh)-4 (Foxj1) mutant mouse due to lack of ependymal cell cilia [144]. The planar cell polarity (PCP) signaling pathway, also known as non-canonical Wnt pathway, has genetic interaction with BBS gene mediated signaling. Both PCP and BBS proteins are colocalized to the basal body and ciliary axoneme, indicating the involvement of their signaling in cilia [133]. Deficiency of mammalian PCP proteins, Celsr2 and Celsr3, the murine orthologs of the Drosophila core PCP gene Flamingo, disrupts the genesis of ependymal cilia, leading to hydrocephalus [145].
Cilia receptor signaling.Polycystin-1 (PC-1), the product of the PKD1 gene, also plays some roles in ciliary function or signaling. Deficiency of PC-1 in ependyma and CP results in dysfunctional cilia and hydrocephalus [146]. β8-integrin transcripts are localized in periventricular cells of the neuroepithelium in the brain, and integrin-β8-deficient mice have characteristics of hydrocephalus [147]. Another recent study reported that the disruption of β1-integrin–matrix interactions in the brain through the injection of β1-integrin antibodies generates ventricular pressure, leading to hydrocephalus development in rats [148]. OPHN1 (oligophrenin1), a RhoGAP protein, binds to the cellular membrane through interaction with phosphoinositides. Ophn1-deficient mice developed hydrocephalus [149].
Ciliopathies are commonly associated with the SCO defect in the pathogenesis of hydrocephalus; thus primary ciliary dyskinesia–associated aqueductal stenosis should be considered as a possible cause for congenital hydrocephalus [150]. Stumpy is a conditional deficient mouse of B9d2 (B9 protein domain 2) in brain and kidney with perinatal hydrocephalus. B9d2 protein, a cellular interacting partner of γ-tubulin, is localized with ciliary basal bodies along ciliary axonemes, suggesting that it plays a role in ciliary axoneme extension [151]. Mutant mice with autosomal recessive congenital hydrocephalus caused by deficient cilia were also found in Ulk4, Nme5, Nme7, Kif27, Stk36, Dpcd, Ak7, and Ak8 gene knockout lines [152].
Deficient water channels
Water channel aquaporin-1 (AQP1), which is expressed at the apical membrane of CP, facilitates CSF production and balance. Membrane-bound AQP1 was reduced following kaolin-induced hydrocephalus, suggesting that CSF accumulation is rooted in AQP1 inhibition or down-regulation in hydrocephalus [153]. Aquaporin-4 (AQP4) is also highly expressed in ependymal cells in brain. Aqp4-null mice developed progressive obstructive hydrocephalus as a consequence of accelerated ventricular enlargement and elevation in intracranial pressure (ICP) due to accumulation of CSF [154]. However, another study found that AQP4-deficient mice did not produce any hydrocephalus or hydrocephalus-like abnormalities in the brain [155]. Interestingly, increased AQP4 expression was observed in congenital hydrocephalus in the HTX rat [156] and kaolin-induced hydrocephalus in Sprague-Dawley rats while no changes in AQP1 or AQP9 were observed [157]. Up-regulated AQP4 expression was interpreted as a compensatory response to maintain water homeostasis in hydrocephalus [154,157]. Another report indicates that AQP4 levels are significantly altered in a spatiotemporal-dependent manner in kaolin-induced hydrocephalus, suggesting the total amount of AQP4 in the brain is irrelevant to the pathogenesis of hydrocephalus [158]. While the role of AQP1 as a regulator of CSF production is proposed in the pathophysiology of hydrocephalus [159], the role of AQP4 in the pathophysiology of hydrocephalus is still debatable [160–162]. Further research is needed to clarify these controversial findings.
Disrupted apoptotic pathways
Precisely controlled apoptosis is essential for normal brain morphogenesis during development [163]. The HTX fetus demonstrates secondary closure of the aqueduct in the perinatal period [164]. This secondary closure of the aqueduct in HTX rats is believed to be due to retrograde degeneration of the thalamus caused by apoptotic cell death [165,166] and failure of cell proliferation, indicating the involvement of altered apoptotic and biogenic events [167,168]. ASPP2 enhances the apoptotic function of p53 [169], and ASPP2-null pups develop hydrocephalus [170]. Caspases (cysteine-containing, aspartate-specific proteases) play key roles in apoptotic signaling in neuronal apoptosis. Hydrocephalic phenotypes have been reported in both caspase-3 and caspase-9 null mice, while a more severe phenotype was observed in the caspase-9 null mice [171,172]. Fas is a member of the tumor necrosis factor/nerve growth factors. In a clinical study, an increased level of the soluble form of the anti-apoptotic regulator Fas (sFas) in the CSF of infants with hydrocephalus was observed, while no difference was found in activated caspase 3 [173]. Apoptotic protein, Apaf1 (human homologue of Caenorhabditis elegans CED-4), plays a central role in the caspase-9-dependent activation of caspase 3 through mitochondrial pathways in embryonic brain. Apaf1-deficient mice show enlarged brains caused by hydrocephalus [174,175]. FGFR3 is a member of membrane tyrosine kinases, fibroblast growth factor (FGF) receptors. FGFR3 affects brain size by modulating neuronal cell proliferation and apoptosis during embryonic development. Mice with the K644E kinase domain mutation in Fgfr3 showed enlargement of the brain [176], while the same mutation in human patients results in neurological anomalies during embryonic development including hydrocephalus [177,178].
Neural tube defects
A longitudinal study on the family history in humans indicates that uncomplicated CHC has a genetic base akin to neural tube defects. The two conditions, at least in a large proportion of cases, might be caused by the same genetic etiology [179,180]. Many cases of CHC associated with neural tube defects have been reported [181–189]. Likewise, 20% of neural tube defects were found to be associated with CHC [190]. Surprisingly, one report found that there is not an obvious additional genetic risk for neural tube defects for CHC patients [191].
Altered immune response
Although some studies reported the activation of macrophages and microglia within the brain in animal hydrocephalus models, little is known of the dynamics of immune response in human congenital hydrocephalus. In one study, brain tissue samples of 10 human fetal cases with hydrocephalus and 10 non-hydrocephalic controls were analyzed immunohistochemically with antibodies directed against MHC class II and CD68 antigens, and tomato lectin. Hydrocephalus samples showed focal collections of CD68 and tomato lectin-positive macrophages along the ependymal lining of the lateral ventricles, particularly within the occipital horn. By comparison, brain tissue samples from controls showed few or no ependymal or supra-ependymal macrophages and the few macrophages that were present were not as intensely immunoreactive as in the hydrocephalus cases. The macrophage response detected at the ependymal lining of the ventricles and within the periventricular area in hydrocephalus may be related both to the severity of hydrocephalus and to the age of the fetus [192]. Unexpectedly, hydrocephalic cases also showed focal regions of hypovascularization or alterations in the structure and orientation of capillaries within periventricular areas, compared to controls [192]. CDO is a cell surface protein, a member of the immunoglobulins (Ig), that is highly expressed in the central nervous system. Cdo knockout mice have developmental defects in brain, including hydrocephalus [193]. SOCS7, a member of the suppressor of cytokine signaling (SOCS) proteins, prominently expresses Socs7 in the brain; mice lacking SOCS7 developed hydrocephalus [194].
Unknown causes
Some hydrophilic mice have been well characterized, but the likely underlying causes in those mutant lines are still undetermined. Those hydrophilic mouse lines are caused by genes mutated in Mboat7, Sgk196, and Fzd3 [152]. Hif-1α, a hypoxia inducible factor response to low oxygen condition, is expressed in the developing brain and contributes to brain development. Disruption of the mouse Zic5 gene, a zinc finger protein, results in hydrocephalus [195]. Neural cell-specific ablation of the Hif-1α gene in mice results in hydrocephalus [196]. Mice with homozygous mutation of molecular cues involving neuronal development, such as NP2 (neuropilin-2) [197], NfiA [198] and NfiX [199] (nuclear factor Ia and nuclear factor Ix), Slc39a4 [200], Otx2 [201], and Myh10 [202], also have a hydrocephalic phenotype.
Enhanced expression during early development
Many cellular mechanisms involved in down-regulation of certain cellular factors, as occur in mutant animal lines, are critical for neural development. Their down-regulation may lead to hydrocephalus. However, many efforts have recently been made to up-regulate certain cellular factors that can also induce hydrocephalus or hydrocephalus-like characteristics, including enlarged ventricles, thinned cortex, agenesis of the corpus callosum, and significantly reduced subcommissural organ in animal models, indicating that elevated expressions of these cellular factors are important parts of the pathogenesis of hydrocephalus.
Kruppel-like factor 4 (KLF4) is a member of the KLF family of transcription factors which are highly expressed in mouse embryonic stem cells (ESCs) and during the early phases of mouse development [203]. Mice with increased expression of KLF4 in neural stem cells (NSCs) and NSC-derived ependymal cells had elevated glial fibrillary acidic protein (GFAP) expression and astrocyte hypertrophy accompanied by defective ventricular cilia, resulting in hydrocephalus [204]. Overexpression of an X-linked transcription factor, Sox3, which is expressed in the murine SCO throughout its development and in the mature organ, disrupts the development of the SCO primordium through inhibition of diencephalic roof plate identity without inducing programmed cell death, therefore generating congenital hydrocephalus [100]. Mice overexpressing the human PAC1 receptor, which is selectively activated by the neuropeptide PACAP, and expressed predominantly in the brain during early development of the CNS, showed marked reductions in the cerebral cortex, corpus callosum, and SCO together with decreased neuronal proliferation and accelerated apoptosis, and shorter, inefficient and disorganized cilia [205]. Those combined defects likely result in reduced CSF flow within the cerebral aqueduct, thereby promoting hydrocephalus [206]. En1 (Engrailed 1), a homeodomain-containing protein that is an early target of Wnt1 signaling during embryonic development, regulates the differentiation of the CP, the main site of production and secretion of CSF. Ectopic expression of En1 gene induced prenatal hydrocephalus in mouse. Ectopically expressed En2 gene in the developing chick embryo developed an identical phenotype to hydrocephalus [207]. Diazepam binding inhibitor (DBI) is a negative allosteric modulator of GABAA receptors; higher DBI levels have been associated with multiple neurological conditions. A hydrocephalic phenotype was developed in the transgenic mouse line with 6-fold higher expression levels of Dbi [208]. Transforming growth factor β (TGF-β) serves broad regulatory roles through its modulation of cell growth and differentiation. The development of severe hydrocephalus was found to be associated with high levels of TGF-β1 mRNA expression in the brain of a transgenic mouse line [209].
Ectopically overexpression of certain cellular factors can induce a phenotype of hydrocephalus, indicating that these cellular factors are involved in the signaling pathways, which when perturbed (could be caused by the loss of function of one of the upstream suppressors in the signaling pathway), leads to pathogenesis of hydrocephalus. Current known hydrocephalic animal models caused by overexpression of certain genes are described in Table 4.3.
Table 4.3 Current known hydrocephalic animal models caused by overexpression of certain genes
Conclusion
Genetic studies in animal models have started to open new ways for understanding the underlying molecular pathophysiology of hydrocephalus. Based on data from animal models, current understanding of the pathophysiology of congenital hydrocephalus is that CHC is the result of abnormal brain development which sequentially leads to disrupted CSF secretion, circulation, absorption, and drainage [210].
Ironically, in contrast to the exciting research advances in genetic studies in animal models, human hydrocephalus genetic research has lagged far behind. In the first National Institutes of Health (NIH)-sponsored research conference on hydrocephalus (Bethesda, 2005), the topic “genetic cause” was “very fortunately” added to the future research tasks at the last minute. Amazingly, in the third NIH-sponsored research conference on hydrocephalus (Seattle, 2013) entitled “Opportunities in Hydrocephalus Research: Pathways to Better Outcomes,” the very first session was about the genetic causes of hydrocephalus. This dramatic change partly reflects our current understanding, rapid research progress, and changing focus on the molecular etiology of hydrocephalus.
In summary, the pathological evidence of hydrocephalus clearly indicates that impaired and abnormal brain development in the early development stage is caused by altered neural cell fate and perturbed regulation of cellular events due to altered molecular cues during development. The abnormal brain development subsequently leads to the accumulation of CSF in cerebral cavities. All these cellular and developmental events are driven by certain genetic determinants, which eventually lead to congenital hydrocephalus accompanied by possible secondary inflammatory reaction and neurovascular pathogenesis.
References


















































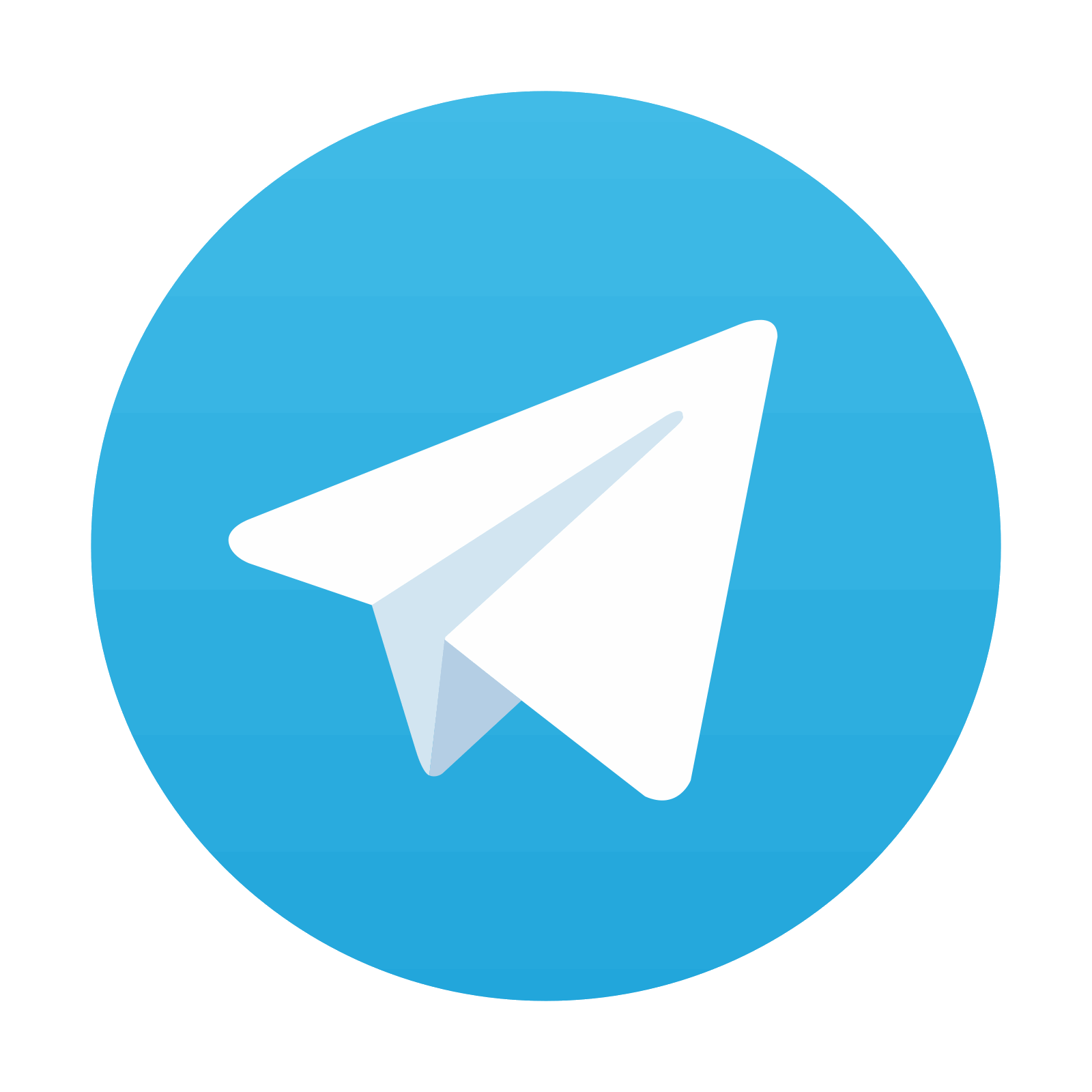
Stay updated, free articles. Join our Telegram channel
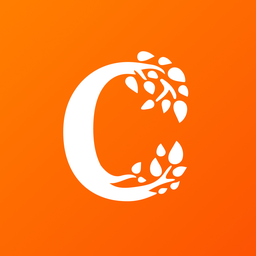
Full access? Get Clinical Tree
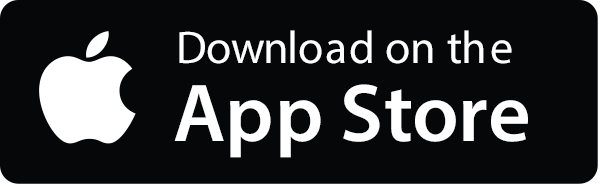
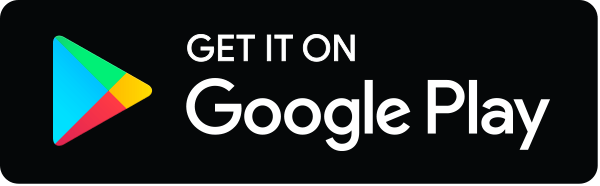
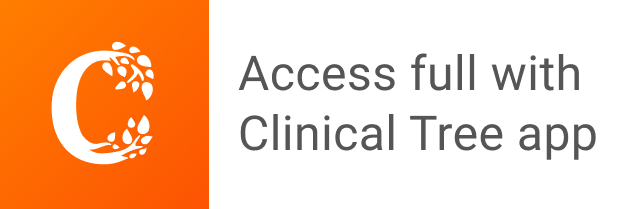