4 Magnetic Resonance Imaging–Based Navigation
Abstract:
Magnetic resonance imaging (MRI) provides high-resolution imaging of soft tissues via the excitation of hydrogen nuclei placed within a large external magnetic field. By varying scanning parameters, different aspects of the anatomic area of interest can be emphasized. Advantages over computed tomography include improved soft-tissue resolution and lack of ionizing radiation; however, these are balanced by increased image acquisition times and need for RF shielding. MRI can be integrated into surgical workflows in several ways: (1) coregistration of a preoperative MRI with intraoperative imaging, (2) colocation of the MRI suite and surgical suite (or gantry-mounted MRI scanners that can enter/exit the surgical field), and (3) performance of surgery within an open bore or double-donut scanner using MR-compatible instruments and equipment. Cost, engineering, and operative time constraints have largely limited utilization of true intraoperative MRI to the treatment of intracranial pathology and spine tumor resection. However, as the demand for minimally invasive surgery grows and technology improves, the role of MRI-based surgical navigation will continue to evolve.
4.1 Introduction
Magnetic resonance imaging (MRI) utilizes nuclear magnetic resonance spectra produced by radiofrequency (RF) excitation of tissue hydrogen nuclei within a strong external magnetic field to generate detailed, volumetric imaging representations of the target structures. It can provide unparalleled soft-tissue image quality for defining normal anatomic structures as well as detecting and delineating pathology. By varying scanning parameters, different output variables (anatomic detail, tissue edema, neural structures, etc.) can be emphasized. Fundamentally MRI differs significantly from fluoroscopy, plain film, and computed tomographic (CT) scanning which all utilize the attenuation of ionizing radiation as it passes through the target tissue to generate an image. In addition to superb image quality, images can be captured independently along multiple axes (as compared to CT where coronal and sagittal images are generated from the natively acquired axial images) with no exposure to ionizing radiation.
Image quality in MRI is a function of signal-to-noise ratio, and in general, better images can be obtained with larger external magnetic fields. Most modern MRI scanners use a cooled superconducting magnet to generate a magnetic field with a strength ranging from 1.5 to 3.0 Tesla (for comparison, Earth’s magnetic field at the surface is 25–65 microteslas) which necessitates exclusion of ferromagnetic materials from proximity to the system. Generated RF signal reception can be improved by proximity of the receiver coils to imaged structures, frequently by use of anatomic region–specific coils (i.e., shoulder or knee) placed on the body surface. Minimization of background noise can be accomplished by utilizing an RF-shielded environment for signal acquisition.
Given some of the physical constraints associated with MRI, real-time incorporation of MRI into surgical workflow presents technical and engineering challenges not encountered with fluoroscopy, intraoperative CT scanning, or visual/infrared navigation. MRI-based navigation in spine surgery can roughly be broken into three different genera: (1) Preoperative MR images are coregistered with intraoperative CT scanning or ultrasound and/or input into an image-based navigation or robotics system. (2) Surgery is performed in an operative suite collocated with an MRI suite allowing for intraoperative transfer of the patient from a traditional operating room (OR) to the MRI scanner and back. (3) True intraoperative MRI, with creation of a surgical field in a double-donut or open bore MRI scanner allowing for near real-time imaging during surgery.
MRI-based navigation allows for surgeon “visualization” of surgical instruments in relation to normal and pathologic anatomy, an effect which is most pronounced where there are narrow approach corridors or where local anatomy precludes dissection and direct visualization. Much of the early literature on the use of MRI in surgery reflects this and describes use of the technology to address intracranial pathology. The goals of this chapter are to provide a background on MRI technology and describe the development of MRI-based surgical navigation, and how it is currently utilized in spine surgery.
4.2 Magnetic Resonance Imaging Basics
MRI has gained widespread use since its introduction as a medical diagnostic tool in the late 1970s. It allows for excellent soft-tissue definition and contrast and can be used to define and distinguish normal anatomy from pathological processes and their sequelae. MRI, as medical imaging modality, emerged from nuclear magnetic resonance (NMR) spectroscopy, a technology developed in the 1940s.
NMR spectroscopy was originally developed as a research tool which utilized the behavior of certain types of atomic nuclei within a strong magnetic field to provide information about the atomic and molecular structure of studied compounds. The resolution of NMR spectroscopy is such that it can distinguish not only between different functional groups in organic compounds but also between identical functional groups which have different neighboring atoms. 1 , 2 , 3
MRI and NMR both rely on a physical property of certain atomic nuclei, called spin, which is the result of interactions between the subatomic particles, namely, gluons and quarks, which compose protons and neutrons. Depending on the number and ratio of protons and neutrons, atomic nuclei may possess spin values from 0 to 8 in half-integer increments. For the purposes of most medical MRI, the abundant hydrogen nucleus, composed of a single proton with spin value ½, is used.
A charged, spinning proton generates a magnetic field parallel to the axis of rotation, and is often stylized as a small bar magnet (Fig. 4‑1 a). Under normal circumstances, the spin axes of protons within tissue are not coordinated (Fig. 4‑1 b); however, when placed within a large external magnetic field (such as the bore of an MRI scanner), designated as B0, the spin axis aligns with the north–south (longitudinal or z) axis of the external magnetic field, B0 (Fig. 4‑1 c). Radiofrequency (RF) energy can then be applied at the resonant frequency of nuclear precession,* which tips the spin axis away from the longitudinal axis of the external field. Typically, RF energy is applied to tip the spin axis 90 degrees to the longitudinal axis, into the xy or transverse plane (Fig. 4‑1 d). (*The resonant frequency of precession, f, is determined by the Larmor equation: f = γB0, where γ is a constant, the gyromagnetic ratio [characteristic of each type of nuclei], and B0 is the main magnetic field strength.)

Cessation of the external RF energy pulse allows time-dependent relaxation of the nuclei spin axes back to their resting state parallel to the longitudinal axis of B0 (Fig. 4‑1 e). This time-dependent relaxation is tissue specific, and differences in relaxation times can be used to differentiate between tissue types. 4 , 5 , 6 , 7 , 8 During relaxation, the nuclei precess (or wobble, like a child’s top) around the longitudinal/z-axis defined by B0. As the nuclei precess, they continue to generate small magnetic fields parallel to their internal spin axis, and these fields can be resolved into z and xy components: when all spin axes are aligned with B0, the z-component of the magnetic field is maximized, and the xy-component is minimized. Conversely, after an RF pulse that tips the spin axes into the transverse plane, the z-component is minimized, and the xy-component is maximized. Imaging sequences that emphasize differences in the longitudinal (z-axis) component of relaxation are described as T1 weighted (Fig. 4‑1 f), while imaging sequences that emphasize differences in transverse plane decay are described as T2-weighted (Fig. 4‑1 g).
Signal generation occurs because precessing nuclei are functionally small, spinning magnets: their precession induces small electrical currents in receiver coils. These induced currents encode information about the structural arrangement and composition of the imaged tissue and are used to generate the visual representation of the imaged structures. 4 , 5 , 6 , 7 Manipulation of imaging parameters, RF pulse sequences, and processing algorithms allows different aspects to the imaged structures to be emphasized; most frequently in spine surgery, fat-suppressed T2-weighted images are used to identify pathologic processes and provides good contrast between cerebrospinal fluid (CSF; hyperintense) and disk material (hypointense; Fig. 4‑2). For oncologic or infectious workups, T1-weighted images obtained before and after administration of intravenous gadolinium contrast can be useful in identifying the extent of tumors and defining cystic structures.

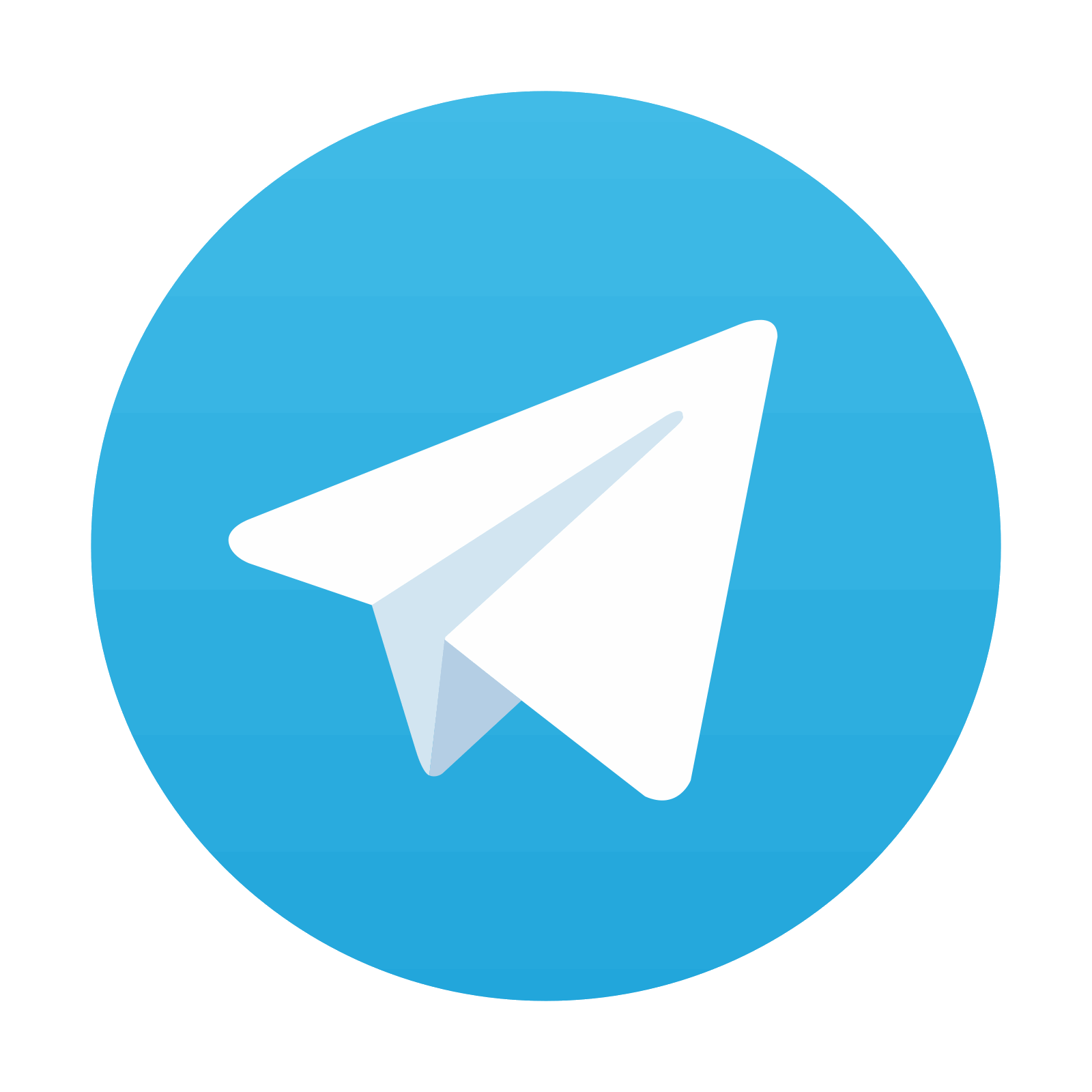
Stay updated, free articles. Join our Telegram channel
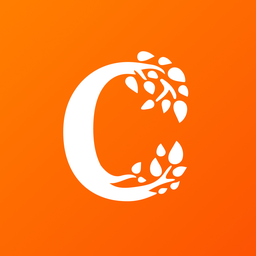
Full access? Get Clinical Tree
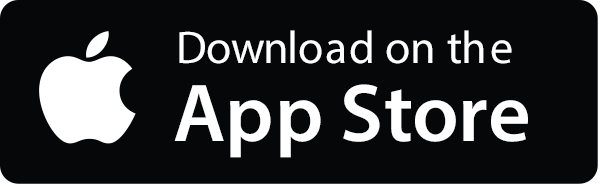
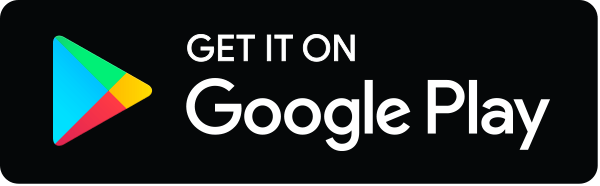