6 Biomechanics of the Unstable Craniovertebral Junction
From a bioengineer’s perspective, biomechanics of the spine involves an understanding of the interaction among spinal components to provide the desired function in a normal person. Thereafter, one needs to analyze the role of these elements in producing instability. Abnormal motion may be because of external environmental factors the spine is subjected to during activities of daily living (e.g., impact, repetitive loading, and lifting), degeneration, infectious diseases, injury or trauma, disorders, and/or surgery. Furthermore, the field of spinal biomechanics encompasses a relationship among conservative treatments, surgical procedures, and spinal stabilization techniques. This chapter deals with the biomechanics of the unstable craniovertebral joint and the common fixation procedures.
Role of Environmental Factors in Producing Instability/Injury
High-speed impact loads that may be imposed on the spine are one of the major causes of spinal instability in the cervical region, especially in the upper region. To quantify the likely injuries of the atlas, Oda and coworkers1,2 subjected upper cervical spine specimens to high-speed axial impact by dropping 3 to 6 kg weights from various heights. The load produced axial compression and flexion of the specimen. Both bony and soft tissue injuries, similar to Jefferson fractures, were observed. The bony fractures were six bursting fractures, one four-part fracture without a prominent bursting, and one posterior arch fracture. The major soft tissue injury involved the transverse ligament. There were five bony avulsions and three midsubstance tears. The study was extended to determine the three-dimensional load displacements of upper cervical spines (C0–C3) in flexion, extension, and lateral bending before and following the impact loading in the axial mode. The largest increase in flexibility due to the injury was in flexion-extension (~42%). In lateral bending, the increase was on the order of 24%; in axial rotation, it was minimal (~5%). These increases in motion are in concordance with the actual instabilities observed clinically. In patients with burst fractures of the atlas, Jefferson noted that the patients could not flex their heads, but they could easily rotate without pain.3
Heller et al.4 tested the transverse ligament attached to the C1 vertebra by holding the C1 vertebra and pushing the ligament in the middle along the anteroposterior (AP) direction. The specimens were loaded with an MTS Bionix (MTS Systems, Inc., Minneapolis, MN) testing device at various loading rates. Eleven specimens failed within the substance of the ligament, and two failed by bone avulsion. The mean load to failure was 692 N (range 220–1590 N). The displacement to failure ranged from 2 to 14 mm (mean 6.7 mm). This study, when compared with the work of Oda et al.,1,5 suggests that (1) AP translation of the transverse ligament with respect to the dens is essential to produce its fracture; (2) rate of loading affects the type of fracture (bony vs. ligamentous) but not the displacement at failure; and (3) even “axial” impact loads are capable of producing enough AP translation to produce a midsubstance tear of the ligament, as reported by Oda and coworkers.
The contribution to stabilization by the alar ligament of the upper cervical spine is of particular interest in evaluating the effects of trauma, especially in the axial rotation mode. Goel and associates,6 in a study of occipitoatlantoaxial specimens, determined that the average values for axial rotation and torque at the point of maximum resistance were 68.1° and 13.6 Nm, respectively. They also observed that the value of axial rotation at which complete bilateral rotary dislocation occurred was approximately the point of maximal resistance. The types of injuries observed were related to the magnitude of axial rotation imposed on a specimen during testing. Soft tissue injuries, such as stretch/rupture of the capsular ligaments and subluxation of the C1–C2 facets, were confined to specimens rotated to or almost to the point of maximum resistance. Specimens that were rotated well beyond the point of maximum resistance also showed avulsion fractures of the bone at the points of attachment of the alar ligament or fractures of the odontoid process inferior to the level of alar ligament attachment. The alar ligament did not rupture in any of the specimens. Chang and associates7 extended this study to determine the effects of the rate of loading (dynamic loading) on the occipitoatlantoaxial complex. The specimens were divided into three groups and tested until failure at three different dynamic loading rates, 50°/second, 100°/second, and 400°/second, as compared with the quasistatic (4°/s) rate of loading used by Goel et al.7 The results showed that at the higher rates of loading, (1) the specimens became stiffer, and the torque required to produce “failure” increased significantly (e.g., from 13.6 Nm at 4°/s to 27.9 Nm at 100°/s); (2) the corresponding right angular rotations (65–79°) did not change significantly; and (3) the rates of the alar ligament midsubstance rupture increased, and that of “dens fracture” decreased. No fractures of the atlas were noted. This is another example of the rate of load application affecting the type of injury produced.
Fractures of the odontoid process of the second cervical vertebra comprise 7 to 13% of all cervical spine fractures. Most published reports involving odontoid fracture use the classification system detailed by Anderson and D’Alonzo.8 They described three types of odontoid process fracture. Type I is an oblique fracture near the superior tip of the odontoid process and is thought to involve an avulsion defect associated with the alar/apical complex. Fracture of the odontoid process at the juncture of the process and vertebral body in the region of the accessory ligaments (type II) is the most common osseous injury of the axis. Fractures of this type lead to a highly unstable cervicovertebral region, commonly threatening the spinal canal, and are often accompanied by ligamentous insult. Many of these fractures result in pseudarthrosis if not properly treated. Type III fractures involve the junction of the odontoid process and the anterior portion of the vertebral body. These fractures are thought to be more stable than the type I and type II fractures. Type III fractures have high union rates owing to the cancellous bone involvement and the relatively high degree of vascularity.8,9
Forces required to produce various types of dens fractures have been documented by Doherty and colleagues,10 who harvested the second cervical vertebra from fresh human spinal columns. Force was applied at the tip of the dens until failure occurred. The direction of the applied force was adjusted to exert extension bending or combined flexion and lateral bending on the tip of the dens. Extension resulted in type III fractures, and the combined load led to type II fractures of the dens. Furthermore, dynamic loading modes are essential to produce midsubstance ligament ruptures as opposed to dens fractures, especially in a normal specimen. Odontoid fractures have been implicated as being the result of high-energy traumatic events. Indeed, there have been numerous accounts as to the events that lead to odontoid fracture. Schatzker et al.9 reported that 16 of the 37 cases they reviewed were due to motor vehicle accidents, and 15 cases were the result of high-energy falls. Clark and White11 reported that all type II (96 patients) and type III (48 patients) fractures they reviewed were attributable to either motor vehicle accidents (~70%) or falls. Alker et al.12 examined postmortem radiographs of 312 victims of fatal motor vehicle accidents. The cohort exhibited 98 injuries of the cervical spine, of which 70 were seen in the craniovertebral junction. The authors, although not quantifying the degree of dens fractures, hypothesized that odontoid fractures were probably due to hyperextension because of the posterior displacement of the fracture pieces.
There is considerable controversy as to the major load path that causes odontoid fractures. A review of the clinical and laboratory research literature fails to designate a consensus on this issue. Schatzker et al.9 reviewed clinical case presentations and concluded that odontoid fractures are not the result of simple tension, and that there must exist a complex combination of forces needed to produce these failures. Althoff13 performed a cadaver study in which he applied various combinations of compression and horizontal shear to the head via a pendulum. Before load onset, the head was placed in neutral, extension, or flexion. The position of the load and the angle of impact, determining the degree of compression with shear, were changed for each experiment. The loading cases in which odontoid fractures were obtained are given in Table 6.1 . The results indicated that an impact in the sagittal plane (anterior or posterior) produced fractures that involved the C2 body (type III). As the force vector moved from anterior to lateral, the location of the fracture moved superiorly, with lateral loading producing type I fractures. This led the author to propose a new hypothesis: impact loading corresponding to combined horizontal shear and compression results in odontoid fractures. Althoff dismissed the contributions of sagittal rotation (flexion and extension) to the production of resultant odontoid fracture.
Mouradian et al.21 reported on a cadaver and clinical model of odontoid fracture. In their opinion, “it seems reasonable to assume that shearing or bending forces are primarily involved.” The cadaver experimentation involved anterior or lateral translation of the occiput, as well as lateral translation of the atlantal ring. In forward loading, the odontoid was fractured in 9 of the 13 cases, with 8 type III fractures and 1 type II fracture. The lateral loading specimens evidenced similar patterns of odontoid fracture regardless of the point of load application (on the occiput or on the atlas). In 11 specimens, lateral loading resulted in 10 type II fractures and 1 type III fracture. The clinical model involved reviewing 25 cases of odontoid fracture. The authors reported that 80% of these cases resulted from flexion or flexion-rotation injuries. They pointed out that the clinical data do not reflect the lateral loading cadaver experimentation results. In fact, they state that “a pure lateral blow probably did not occur in any [clinical] case.” However, their clinical data indicated that the remaining 20% of the odontoid injuries could be ascribed to extension injuries. Technical diffculties precluded cadaver experimentation of this possible mechanism.
Experimental investigations dealing with the pathogenesis of odontoid fractures have failed to produce a consensus as to the etiology of these fractures. These findings may actually reflect the diversity of causal mechanisms, suggesting that various mechanical factors are coincident in producing these fractures. It is difficult to discern if this is the case or if this is due to the inhomogeneity of cadaver experiment methodology. That is, some of the boundary and loading conditions used by the surveyed studies are vastly different and have produced divergent results. In addition, the anatomical variants of the craniovertebral osteoligamentous structures could also be integral to the cadaver study outcomes. The purpose of the study undertaken by Puttlitz et al.22 was to use the finite element method, in which the loading and kinematic constraints can be exactly designated, for elucidating the true fracture etiology of the upper cervical spine ( Fig. 6.1 ). Previous laboratory investigations of odontoid process failure have used cadaver models. However, shortcomings associated with this type of experimentation and the various loading and boundary conditions may have influenced the resulting data. Utilization of the finite element method for the study of odontoid process failure has eliminated confounding factors often seen with cadaveric testing, such as interspecimen anatomical variability and age-dependent degeneration. This has allowed the authors to isolate changes in complex loading conditions as the lone experimental variable for determining odontoid process failure.
There are many scenarios that are capable of producing fracture of the odontoid process. Force loading, in the absence of rotational components, can reach maximum von Mises stresses that far exceed 100 MPa. Most of these loads are lateral or compressive in nature. The maximum stress obtained was 177 MPa due to a force directed in the posteroinferior direction. The net effect of this load vector and its point of application, the posterior aspect of the occiput, is to produce a compression, posterior shear, and extension because of the load’s off set from the center of rotation. This seems to suggest that extension and compression can play a significant role in the development of high stresses, and possibly failure, of the odontoid. The location of the maximum stress for this loading scenario was in the region of a type I fracture. The same result, with respect to lateral loading, was obtained by Althoff.13 However, he dismissed the contribution of sagittal plane rotation to development of odontoid failures. The results of this study disagree with that finding. Posteroinferior loading with extension produced a maximum von Mises stress in the axis of 226 MPa. As stated previously, the load vector for this case intensifies the degree of extension, probably producing hyperextension. The addition of the extension moment did not change the location of the maximum stress, still identifiable in the region of a type I fracture. Mouradian et al.’s21 clinical study suggested that almost 20% of the odontoid fracture cases they reviewed involved some component of extension. The involvement of extension in producing odontoid process failures can be explained by its position with respect to the atlantal ring and the occiput. As extension proceeds, the contact force produced at the atlantodental articulation increases, putting high bending loads on the odontoid process. The result could be failure of the odontoid. Increasing tension of the alar ligaments as the occiput extends could magnify these bending stresses via superimposition of the loads, resulting in avulsion failure of the bone (type I).

Although the finite element model predicted mostly higher stresses with the addition of an extension moment, it showed that, in most cases, flexion actually mitigates the osseous tissue stress response. This was especially true for compressive (inferior) force application. Flexion loading with posterior application of an inferior load vectorally decreases the overall effect of producing extension on the occiput. None of the studies surveyed for this investigation pinpointed flexion per se as a damage mechanism for odontoid failure. The findings of this study supported the lack of evidence of flexion as a causal mechanism for failure. In addition, the data suggested that flexion can act as a preventive mechanism against odontoid fracture.
Once again, the lateral bending results support the hypothesis of extension being a major injury vector in odontoid process failure. Inferior and posteroinferior loads with lateral rotation resulted in the highest maximal von Mises stress in the axis. Lateral loading also intensified the maximal stress in compression, suggesting rotations that incorporate a component of both lateral and extension motion may cause odontoid failures. Many of the lateral bending scenarios resulted in the maximum von Mises stress being located in the type II and type III fracture regions. In fact, the only scenarios that lead to the maximum stress in the type I area were when there was an inferior or posterior load applied with the lateral bending. This is, again, suggestive that the extension moment, produced by these vectors and their associated moment arms (measured from the center of rotation), can result in more superiorly located fractures.
Overall, this investigation has indicated that extension and the application of extension via force vector application cause the greatest risk of superior odontoid failure. The hypothesis of extension as a causal mechanism of odontoid fracture includes coupling of this motion to other rotations. Flexion seems to provide a protective mechanism against force application that would otherwise cause a higher risk of odontoid failure.
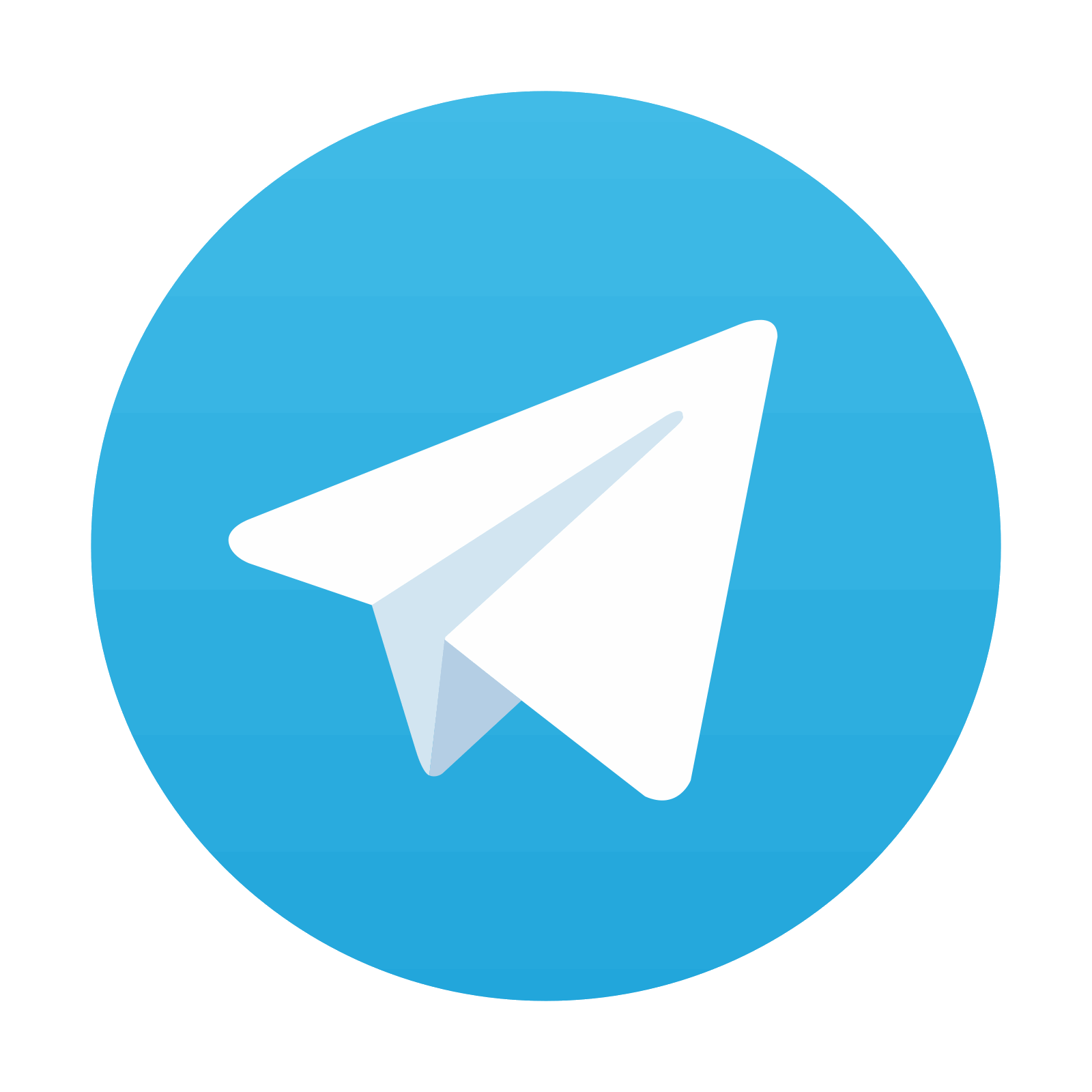
Stay updated, free articles. Join our Telegram channel
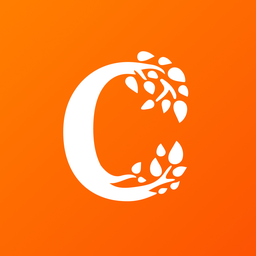
Full access? Get Clinical Tree
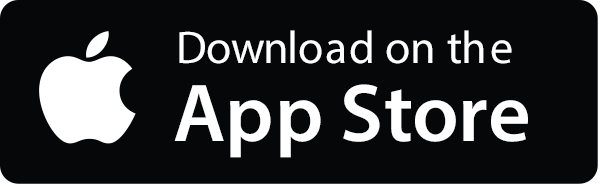
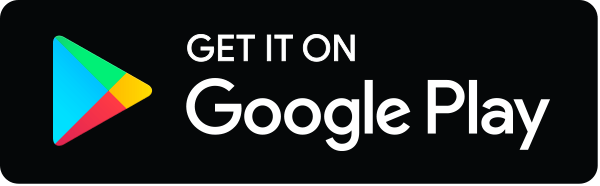