6 Neurophysiology of Identifying Eloquent Regions
Abstract
Neurophysiological monitoring is a useful technique during functional brain mapping (FBM) with and without an identifiable brain lesion. It helps preserve functional integrity of tissue and optimal surgical resection leading to improved postoperative neurosurgical outcome. Examples of noninvasive FBM techniques include scalp electroencephalography, evoked potentials, magnetoencephalography, and transcranial magnetic stimulation. However, these techniques may be limited by their inability to precisely map the brain due to temporal or spatial constraints. Direct electrical stimulation has been the gold standard for FBM and demonstrates utility as a therapeutic tool for aiding resections in, near, or overlying regions of brain imparting eloquent function. Furthermore, a focus on preserving cortical function during FBM has led to additional insight in meticulously evaluating subcortical functional regions to avoid injury to the subcortical structures that lead to neurological deficits. Integrating multiple FBM techniques improves the precision of preserving eloquent brain regions. New noninvasive techniques advance hope of achieving greater safety profiles, better tolerability, and shorter procedure times to precisely outline the structural–functional relationships of an individual’s brain anatomy, to optimize targeted resections and improve long-term functional outcomes.
6.1 Introduction
Resection of brain tumors and other lesions adjacent to eloquent regions constitutes a principal challenge in neurosurgery. Because maximal gross total resection of abnormal tissue is desirable without introducing a postoperative neurological deficit, working knowledge of the brain’s functional topography in and around the lesion is critical. Functional brain mapping (FBM) is routinely accomplished through the integration of various techniques. Noninvasive means to localize structural pathology center on high-resolution brain magnetic resonance imaging (MRI) with an epilepsy protocol. Functional procedures such as electroencephalography (EEG) and magnetoencephalography (MEG) identify physiological integrity of the neuron, tractography reflects function of the axons, functional MRI (fMRI) identifies vascular function, MR spectroscopy analyzes neurochemistry, positron emission tomography (PET) reflects metabolism, single photo emission computed tomography (SPECT) identifies regional blood flow, and Wada testing reflects neuropsychological function.
The human brain is segmented into eloquent and clinically silent regions. Eloquent cortex are areas responsible for essential function in daily life. If removed or injured, a loss of sensory function, paralysis, visual loss, or deficits in language may result. Indispensable regions where irreversible postoperative deficits can occur include primary motor cortex, primary sensory cortex, primary visual area, and anterior and posterior language areas. Other areas where deficits following surgery by virtue of network connectivity may occur but may not be long lasting include operable areas in the primary somatosensory and motor areas, primary auditory cortex, secondary sensory cortex, and basal temporal language area. 1 By contrast, resection of clinically silent areas of brain results in no discernible consequences of function.
Knowledge of the functional capability for brain that is surrounding a lesion is indicated for the resection adjacent to eloquent cortex (Table 6‑1, Fig. 6‑1). This may include surgical resection of a seizure focus, brain tumor, or vascular lesion to cite a few common examples. FBM informs the operating surgeon to determine a safe balance between the extent of resection versus minimizing postoperative neurological deficits from injury to eloquent regions. FBM has evolved from within the boundaries of the operating room to the epilepsy monitoring unit (EMU). Intraoperative FBM may be performed for tumors and immediately prior to surgical resection but has limitations in the practical use of this procedure. By contrast, preoperative epilepsy surgery evaluation is typically performed within the EMU using intracranial electrodes (e.g., subdural, depth, and stereotactic), where the seizure focus is first delineated using noninvasive EEG electrodes. Following placement of intracranial electrodes, elective direct electrical stimulation (DES) of selected electrodes is utilized to identify and map the eloquent regions of brain surrounding the seizure focus. Development of novel FBM noninvasive techniques (e.g., fMRI, transcranial magnetic stimulation [TMS], passive electrocorticography [ECoG], spectral analysis of high gamma activity [HGA], and artificial intelligence/deep brain machine learning) is emerging given their potential to replace conventional methods using DES. 2 However, DES has remained pivotal to FBM as the gold standard for cortical and subcortical FBM since its recognition by Penfield and colleagues. 1 , 3 , 4 , 5 Of note, the Bonini paradox 6 illustrates the dichotomous nature of outlining territories involved in FBM—simpler maps are less accurate though more useful representations of the territory. Techniques used attempt to optimize the balance between accuracy and practicality when contemplating the most suitable technique for FBM.

The goals for this chapter are to provide the reader with an understanding of the variations in clinical neurophysiological techniques to identify crucial neuroanatomic sites serving as eloquent regions. FBM and different neurophysiologic techniques utilizing extra- and intracranial mapping are available to guide better patient outcomes in the hands of an experienced neurosurgical team.
6.2 Indications for Functional Brain Mapping
6.2.1 Epilepsy
Epilepsy surgery aimed at the resection of an epileptogenic foci was an initial area to use clinical neurophysiological techniques in FBM. Resective epilepsy surgery is a standard of care in patients with drug-resistant focal epilepsy and has shown to significantly improve long-term, seizure-free outcomes. 7 Often, areas of epileptogenesis may involve the eloquent cortex and mapping of cortical function is critical during presurgical planning (Fig. 6‑2). FBM has been utilized in the resection of perirolandic and dominant neocortical temporal lobe epilepsy. Remarkably, FBM during epilepsy surgery is distinct from resection of tumors such that FBM is primarily aimed at localizing the epileptogenic zone first, followed by mapping functional areas. Further, epilepsy surgery evaluations commonly occur outside the operating room and within the EMU using scalp video EEG monitoring. When noninvasive techniques fail to localize the seizure onset zone, intracranial electrodes are placed in the operating room and patients are then transferred to the EMU for seizure monitoring with video EEG. Subdural grids (Fig. 6‑3) may be used during intraoperative or extraoperative recording of intracranial EEG to localize the seizure onset zone and map large cortical areas for eloquent brain function. 8 Stereo-EEG monitoring is increasing and as a result electrical stimulation mapping has shown utility as a unique methodology associated with special advantages, disadvantages, and potential safety concerns relative to parameters that are used. 9 EEG technologist and specialized nurses are also present if a seizure were to occur and are responsible for fixing electrode dislodgement after a seizure event, monitor for cerebrospinal fluid leakage or bleeding, and rebandage the head to limit spread of infection. 10 After localizing the seizure focus, intracranial electrodes can then be used to map eloquent regions using DES extraoperatively after several habitual seizures have been recorded to characterize an individual’s epilepsy syndrome for surgical resection or ablation of the epileptogenic zone. 11 When intracranial monitoring (phase II) is required due to discordant information obtained on noninvasive evaluation, 12 DES is typically performed after localizing the seizure onset zone.


6.2.2 Brain Tumors
Primary brain tumors arise directly from the brain parenchyma, while secondary lesions are metastatic from another source. Low-grade gliomas often have a slow clinical course and balancing degree of resection with postoperative functional neurological deficits is essential when tumors occur near or within eloquent brain regions. Low-grade gliomas have been found to contain functional tissue, and therefore, outcome may vary by extent of resection. 13 Postoperative outcome may be severely compromised when blindly resecting infiltrating gliomas without guidance. 14 FBM defines eloquent boundaries to improve surgical outcomes in glioma patients (Fig. 6‑4). In a meta-analysis of 90 reports on resective surgery for supratentorial infiltrative glioma with or without intraoperative DES, late severe neurological deficits were observed in only 3.4% of DES-resected patients, while 8.2% of the non–DES-resected patients demonstrated deficits. 15 Additionally, gross total resection was achieved in a greater number of patients undergoing DES for FBM despite patients having tumor in eloquent regions. 15 As a result, the authors recommend FBM as a standard of care in resecting brain tumors.

Brain metastases are the most frequent intracranial tumors and due to the overall poor prognosis, they should have surgical management individualized when they occur in close proximity or involve eloquent regions of the brain. 16 , 17 Palliative surgery seeks to optimize functional capacity without worsening the existing capacity or introducing new neurological deficits. Thus, localizing the eloquent cortices through a combination of intraoperative mapping techniques with neurophysiological monitoring has been recommended. 18 , 19 In a study of 33 patients with perirolandic metastases, DES was shown to improve surgical planning and resection, as well as spare the eloquent regions by precisely outlining the functional cortical and subcortical structures. 19 Gross total resection of the metastases was achieved in 31 patients (93.9%), and at 6 months follow up, 88.9% had a Karnofsky Performance Scale score greater than 80% and a mean survival time of 24.4 months. 19
6.2.3 Other Brain Lesions
FBM has expanded to include the resection of any brain lesions at risk for postoperative neurological deficits from injury to the eloquent region. These lesions include vascular lesions, abscesses, granulomas, and trauma in addition to others. Resecting the complex interconnecting arteries and veins of arteriovenous malformations (AVMs) located within eloquent cortex is challenging and prognosis is often poor after surgical resection. 20 Due to brain plasticity, reorganization of functional areas may commonly shift from their anticipated location during embryonic development of AVMs. 21 Functional imaging studies have been used to identify surgical landmarks during the preoperative management of cavernous malformation resection. 4 Neurophysiological procedures such as MEG have also demonstrated success in localizing the central sulcus as a reference point when AVMs are located near the motor cortex. 22
FBM techniques may extend beyond just the preoperative planning phase. For example, in a patient with phantom limb pain, fMRI was used to guide the placement of a chronic motor cortex stimulator. 23 PET imaging has been used to visualize brain metabolic activity in an experimental model of heat allodynia. 24 Further, there are various neurostimulation techniques that have been used to restore functional integrity (e.g., epilepsy, Parkinson disease, chronic pain, and obsessive–compulsive disorder) and include deep brain stimulation (DBS), motor cortex stimulation (MCS), responsive neurostimulation (RNS), spinal cord stimulation (SCS), and vagus nerve stimulation (VNS). Further, neurostimulation has also been described as a technique for neural repair of motor function, cognition/memory, and vision. 25 , 26 , 27 Remarkably, functional mapping of the occipital cortex may assist with the development of a prosthesis for the blind using a brain–computer interface, and thus, stimulation of the primary visual cortex using intracortical stimulation may have a role in the treatment of cortical blindness. 28
6.3 Clinical Neurophysiology Techniques
6.3.1 Electroencephalography
EEG records and monitors spontaneous electrical activity of the brain, and can be performed noninvasively by placing scalp electrodes, or invasively, through intracranial electrodes (Fig. 6‑5). EEG measures voltage oscillations from ionic current within the neurons and can be of great utility in the diagnosis of neurological conditions but is especially suited for detecting epileptiform abnormalities and seizures, disorders involving the level of consciousness (stupor and coma), stages of sleep, depth of anesthesia, and when confirming death.

Intracranial EEG or ECoG has been used for studying electrophysiological correlates to functional brain activation (Fig. 6‑6). By contrast to standard scalp EEG recordings, ECoG produces waveforms that are approximately 10 times greater in amplitude, provides higher resolution and spatial information, and has better sensitivity and improved signal-to-noise ratio (high-frequency EEG activity) due to the adjacent proximity of the electrodes to the cortical surface. ECoG is particularly helpful in identifying potentially epileptogenic tissue in patients with drug-resistant focal epilepsy and has been widely used during motor and language mapping, in the pediatric population, and within the EMU setting. 29 , 30 , 31

ECoG has also been used to study event-related dynamics of brain oscillations over a wide range of frequencies and within the somatosensory/motor systems, language networks, and visual and auditory systems. 2 Previously, noninvasive techniques have demonstrated event-related desynchronization/synchronization (ERD/ERS) within lower frequencies, and this observation has extended the use of ECoG in determining event-related responses within much higher gamma frequencies. 9 , 32 Changes in the broadband gamma range (>60 Hz) are of clinical relevance and high-frequency oscillations, including gamma (30–80 Hz), ripples (80–250 Hz), and fast ripples (250–500 Hz), have been observed in various functional brain systems and can be recorded with equipment capable of high sampling rates (Fig. 6‑7). 33 The temporal and spatial localization of high gamma oscillations on passive ECoG are specific to unique activation of functional brain areas that are generated by large populations of neurons produced by a single task. 34

ECoG offers similar precision when compared to DES, but has a greater safety profile, better patient tolerability, and a shorter procedure time. 35 Studies comparing results of sensorimotor mapping from ECoG to those of DES report sensitivities between 0.43 and 1.0 and specificities of 0.72 to 0.94. 36 , 37 , 38 Using ECoG and DES together has several benefits; it reflects the level of wakefulness by monitoring background activity, assesses the effects of anesthesia, verifies the integrity of DES by identifying stimulation-induced artifact, and obtains a recording with after-discharges resulting from overstimulation. Delineating after-discharges helps ascertain that electrographic seizures are not missed or result in provoked seizures due to repetitive stimulation. 39 A study evaluating extraoperative and intraoperative ECoG, DES, and fMRI during mapping of expressive language function reported that integration of multiple functional mapping techniques improved precision of functional localization of the eloquent language cortex. 35 In this study, extraoperative ECoG and DES were both found to be effective in outlining critical functional areas, which was later confirmed using intraoperative high-resolution ECoG. Nonetheless, limitations to ECoG exist. Brain mapping using ECoG either requires a staged procedure or be performed during an awake craniotomy (AC). Longer durations associated with implanted electrodes may significantly increase risk for infection and prolong costs, duration of hospitalization, and delay recovery. 40 , 41
6.3.2 Evoked Potentials
Evoked potential (EP) monitoring has been used diagnostically to localize the central sulcus in patients undergoing surgery near the motor strip. 42 Somatosensory evoked potentials (SSEPs) are normally used to evaluate the integrity of the somatosensory system. The EPs comprise a series of negative and positive waveform deflections. Electrical stimulation of Ia afferents peripheral nerves in the upper (median nerve: Erb’s point/N9, N13, P14, N20) or lower (tibial nerve: lumbar potential, P31, N34, N37) limbs conduct activity through the dorsal columns in the spinal cord and medial lemniscus in the brainstem to the contralateral primary somatosensory cortex. Intraoperative ECoG recording from an electrode array containing at least eight contacts placed across the area anticipated to contain the central sulcus is performed to guide surgical landmarks. The N20 potential is recorded over the somatosensory cortex and P22 potential over motor cortex as far field potentials that are activated following peripheral nerve stimulation to produce a “pseudo-phase reversal” (Fig. 6‑8). The central sulcus lies directly beneath. Additionally, SSEPs may localize sensory deficits, identify silent lesions, and monitor sensory pathway changes during other forms of surgery (e.g., carotid and spinal). 43 Nearly four decades ago, noninvasive brain stimulation, transcranial electrical stimulation (TES), was used as a brief, single, high-voltage electrical current that produced a synchronous motor response eliciting motor EPs from peripheral muscles.

TES uses single- or repetitive-pulse stimulation of the scalp (between 2 and 9) to produce an electrical volley that propagates via the spinal cord to recording electrodes placed over the small muscles in the hand. During TES, high-intensity stimuli are delivered to the scalp in order to stimulate the cortex through an intact skull. 44 The stimulus voltage and current are used at levels far above those necessary to elicit SSEPs and are often used in conjunction during intraoperative monitoring, particularly during spinal surgery. However, some investigators have noted inaccurate results between those obtained from motor evoked potentials (MEP) monitoring and postoperative neurological outcome. 45 , 46
Corticocortical evoked potential (CCEP) may be utilized in assessing language connectivity. CCEP involves stimulating one cortical area while recording an averaged response of a signal that is generated in another area to assess functional interconnections. 47 , 48 In a study of 13 patients, CCEP monitoring was utilized during resection of a tumor located in the dominant cerebral hemisphere, in or around the language-related area. 14 Subdural strip electrodes were placed over the frontal and temporal language areas for recording with potentials that were identified using cortical DES via an adjacent subdural grid. Results indicated that the presence of CCEP responses correlated with the occurrence of postoperative language function, and time to recovery for speech function was significantly associated with changes observed on CCEP as unchanged, decreased, or disappeared when resected at 1.8 ± 1.0 months, 5.5 ± 1.0 months, and 11.0 ± 3.6 months, respectively (p < 0.01). Given that CCEP does not require patient input, monitoring speech function under general anesthesia (GA) was possible without an AC. 49
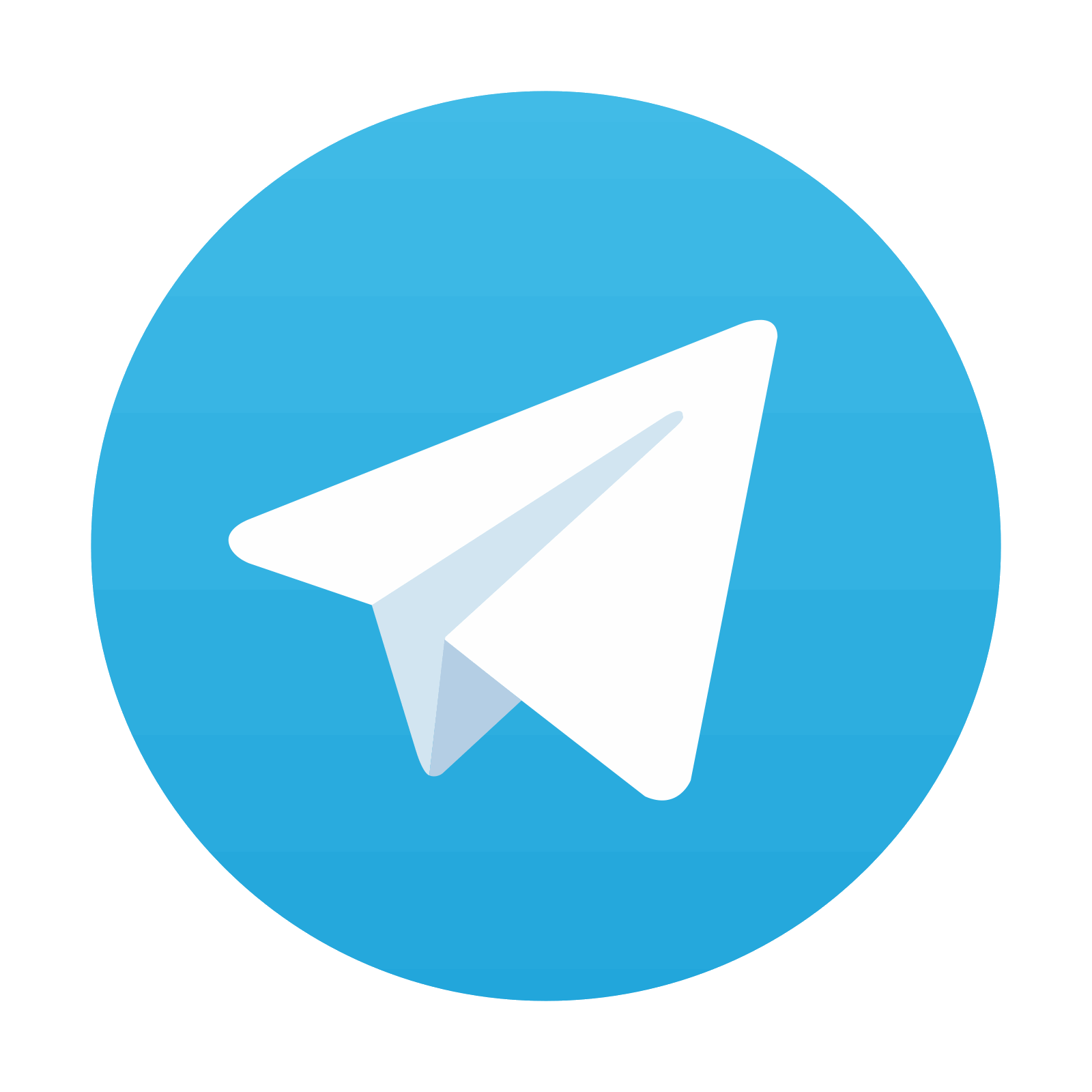
Stay updated, free articles. Join our Telegram channel
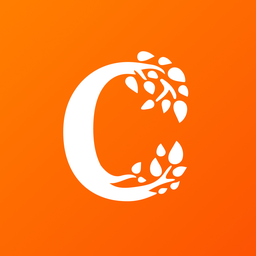
Full access? Get Clinical Tree
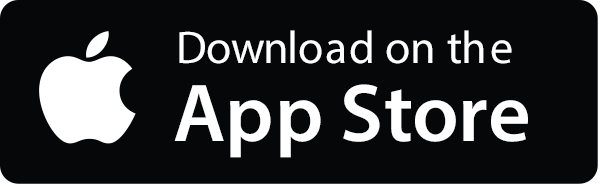
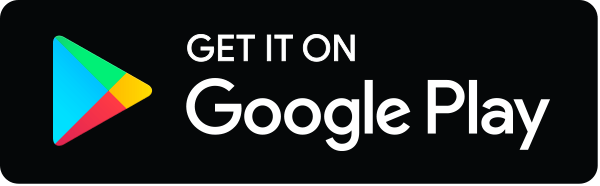
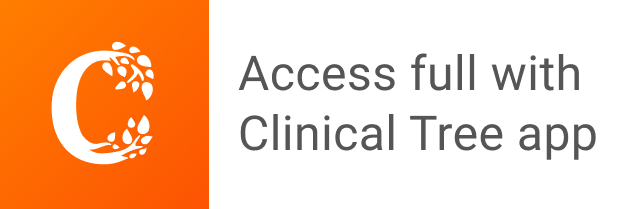