Summary
This chapter covers radiotherapy principles and modern radiotherapy techniques for skull base tumors. (The clinical applications of radiotherapy in skull base tumors are discussed in Chapter 9.) Radiation physics deals with the properties of photon and particle (e.g., proton) radiation and how they are incorporated into radiation treatment planning, whereas radiobiology covers the basis of dose and fractionation, including hypofractionation and ablative dose delivery, and their effects on tumor and normal tissue biology. Advanced treatment approaches such as intensity-modulated radiation therapy, volumetric arc therapy, carbon ion therapy, intensity-modulated proton therapy, and stereotactic radiosurgery allow highly conformal dose distribution with a steep dose gradient beyond the target volume, thereby reducing the risk of normal tissue damage and widening the therapeutic window. To guide precise and accurate treatment delivery, image-guided radiotherapy incorporating concepts of motion management, target volume delineation, and tracking is discussed.
8 Radiotherapy for Skull Base Tumors: Principles and Techniques
8.1 Introduction
Fractionated radiation therapy and stereotactic radiosurgery (SRS) represent two conceptually different approaches to using ionizing radiation to treat tumors. Fractionated radiation relies on the radiosensitivity difference of the tumor relative to the surrounding normal tissue and is less reliant on precise target localization than radiosurgery is. The concept of fractionation is based on classical radiobiology principles and has been validated clinically in the treatment of head and neck cancers. SRS aims to deliver an ablative dose to the target with a steep dose fall-off outside the target so as to preserve the surrounding normal tissue. SRS was developed by Lars Leksell, a Swedish neurosurgeon, based on the concept of delivering a single high dose of radiation to an intracranial target localized via stereotaxy with high precision and accuracy. Advancements in medical imaging have enabled improved visualization of the tumor and the surrounding anatomy. Corresponding advances in radiation treatment planning and delivery systems have enabled the precise delivery of highly conformal radiation doses to the target so as to minimize collateral damage to adjacent normal organs and preserve normal function.
8.2 History and Principles of Radiation Oncology
The history of radiation therapy began with the discovery of X-rays in 1895 by German physicist Wilhelm Conrad Roentgen. The first documented therapeutic use of radiation came in 1897, when Professor Leopold Freund demonstrated before the Vienna Medical Society the resolution of a hairy mole after a single exposure to low-dose X-rays. A shift toward fractionated (i.e., involving division of the dose of radiation over a number of treatments) radiotherapy was led by the pioneering work of scientists in Paris, France, who explored the effects of radiation on the testes of rams. They showed that sterilization of the testes in a single dose caused extensive skin sloughing from the scrotum but that when the same dose was fractionated over several weeks, sterilization was achieved with minimal skin damage. This work would eventually establish fractionation as the major form of therapeutic radiation. Other milestone developments in the field included the work of Nobel laureate Hermann Joseph Muller on the genetic effects of radiation, published in 1927, and research performed in the wake of atomic weapons use on Hiroshima and Nagasaki in World War II. Together, these milestones elucidated the biological effects of radiation exposure and radiation-induced carcinogenesis and led to the development of the cell survival curve.
Modern radiation therapy plays a major role in the treatment of skull base neoplasms, both as curative treatment and to palliate tumor-related symptoms. Curative radiotherapy can be delivered as adjuvant therapy, to reduce the risk of tumor recurrence after surgery, and as definitive therapy in which radiation is the primary treatment modality. The ultimate goal of radiotherapy is to achieve 100% tumor control probability (TCP) and thereby prevent local or regional recurrence. Because nearby normal tissues are also exposed to radiation, the normal tissue complication probability (NTCP) increases. The difference between TCP and NTCP is the therapeutic window (Fig. 8.1).

For skull base tumors, this therapeutic window can be narrow, because the tumor is in proximity to critical neural and optic structures. Technological advances in radiation therapy, such as conformal radiotherapy, image-guided radiation therapy (IGRT), and SRS, aim to widen this therapeutic window. Radiation physics and radiobiology provide the founding principles of modern radiation delivery methods and understandings of fractionation and of biologic tissues’ response to radiation. In addition to discussing the role of radiotherapy in the management of skull base tumors, a goal of this chapter is to provide the reader with an opportunity to become familiar with the terminology and concepts used in radiation oncology. In the following sections, we briefly introduce several commonly used terms in radiation oncology, with further details provided throughout the chapter.
8.2.1 Target Volumes
There are three main target volumes in radiation treatment planning (Fig. 8.2). The gross tumor volume (GTV) is the visualized gross disease as defined by physical examination and imaging studies. High-quality radiographic imaging is essential for accurate contouring of tumor. The clinical target volume (CTV) is the GTV plus an additional margin to account for microscopic extensions and subclinical disease spread beyond the visualized tumor. For example, pathology studies have shown that in non–small cell lung cancer, a 6-mm CTV margin is required to adequately cover microscopic extension in squamous cell carcinomas, whereas an 8-mm margin is needed for adenocarcinomas.1 The planning target volume (PTV) is the CTV plus an additional margin to account for uncertainties in treatment planning and delivery, patient setup and positioning, and tumor motion. The PTV, which is largely a geometric expansion based on these uncertainties, is designed to ensure that the intended radiation field covers the entire CTV with each fraction. The organ at risk (OAR) is the volume of a vital organ close to the tumor that should be spared to reduce treatment toxicity.

8.2.2 Alpha/Beta Ratio
The alpha/beta (α/β) ratio is a measure of the radiosensitivity of a tissue type using the ratio of two parameters to describe how a tissue or cell responds to radiation as a function of the dose (Fig. 8.3). The α component is a measure of the intrinsic radiosensitivity of the cell. The β component represents the repairable portion of radiation damage. Tissues that have small α/β ratios are generally less radiosensitive at standard fractionation doses of 2 Gy (i.e., larger β) and imply a large repair capacity (i.e., smaller α). These tissues have a larger sparing effect at standard doses and are typically observed in late-responding tissues, such as nervous tissue. Tissues that have high α/β ratios either imply a poor repair capacity (i.e., smaller β) and/or are more radiosensitive (i.e., larger α). They are typically observed in early-responding tissue, such as skin and mucosal epithelia and most malignant tumors.

8.2.3 Linear Energy Transfer and Relative Biological Effectiveness
Linear energy transfer (LET) is the rate of energy deposited by the charged particles of ionizing radiation. Relative biological effectiveness (RBE) is a term commonly used to compare different types of radiation and their biological effectiveness; it is the RBE of test radiation when compared with a reference radiation that produces the same effect. In most cases, the test radiation refers to protons, neutrons, and carbon ions and the reference radiation is photons or gamma rays. Proton and carbon ion radiation have a higher RBE than photons, because they cause more biological damage at a given dose. As a general rule, radiation having higher LET will also have a higher RBE and thus cause more biological damage.
8.2.4 Fractionation and Sparing Effect
As outlined earlier, fractionation is the concept of breaking up the total dose into smaller daily fractions to give normal tissues time to repair (Fig. 8.4). This approach exploits the radiosensitivity difference between rapidly growing tumor cells and most normal tissues. Thus, when the same radiation dose is given in multiple fractions separated by sufficient time for cell repair (~ 6 h for twice-daily treatments and 24 h for daily treatments), sparing of the tissue (reduction of damage) occurs in a degree depending on the type of radiation used, the dose per fraction given, and the type of tissue irradiated. Standard (conventional) fractionation doses of 1.8 to 2.0 Gy are typically used to allow for effective sparing of normal tissue.

8.2.5 Altered Fractionation
Altered fractionation refers to various fractionation schemes developed over the past few decades that differ from standard fractionation. Hyperfractionation is the breaking up of standard fractionation doses into smaller doses given with higher frequency, with the goal of further exploiting the differences in sensitivity between tumors, which typically have a higher α/β ratio, and normal tissue, which typically has a lower α/β ratio (most parenchymal tissue). The intent of this approach is to lower late toxicity risk in patients who receive conventional radiotherapy. Accelerated fractionation, or the shortening of overall treatment time to address the risk of accelerated tumor repopulation that occurs after initiation of oncologic therapy, is often achieved without altering total dose or dose per fraction. A common accelerated fractionation schedule used in head and neck squamous cell carcinoma (HNSCC) is the Danish Head and Neck Cancer Study Group (DAHANCA) regimen of six fractions per week instead of five, which shortens overall treatment time and improves tumor response.2 Hypofractionation involves increasing the dose per fraction (usually > 3 Gy per day) above the standard fractionation dose. Doing so reduces the number of fractions and shortens the overall course of treatment, results that are logistically and financially favorable. Larger dose per fraction increases the probability of overcoming intrinsic cell resistance and repair capabilities. The increased use of hypofractionation coincides with an increase in stereotactic radiation therapy (SRT) use.
8.2.6 Biologically Effective Dose
The biologically effective dose (BEDn) is a summation of the true biological dose delivered as a function of dose per fraction, total dose, and associated α/β ratio (n). Often used to determine the optimal dose needed to achieve the same biological effect when deviating from standard fractionation,3 , 4 it can be used to increase the TCP, and thus improve tumor control without increasing toxicity, or decrease the NTCP, thereby decreasing treatment complications without compromising tumor control. For example, for HNSCC in which we assume an α/β ratio of 10, the BED10 for 70 Gy in 35 fractions is 84 Gy. To achieve a similar BED in 5 fractions, 8.9 Gy per fraction would be required.
8.2.7 Bragg Peak
The Bragg peak, a term common in particle therapy, is the depth in tissue at which charged particles release most of their energy to cause a biological effect. The spread out Bragg peak (SOBP) is a broader peak, composed of multiple Bragg peaks, that covers the entire tumor. The SOBP is produced using different energies and/or tissue attenuators.
8.2.8 Biologically Equivalent Dose
The dose equivalent, which is used to compare charged particles with photons, is the physical dose corrected for biological effectiveness. Different types of particle radiation with differences in LET have different RBE when compared with photons. As a result, the physical dose, in Gray (Gy), of the particle radiation must be multiplied by its RBE and is expressed as Gy (RBE). In many proton studies, the term cobalt Gray equivalent is used to refer to a proton RBE of 1.1 with respect to cobalt-60. Note that the biologically equivalent dose is not the same as the BED; biologically equivalent dose is calculated in 2 Gy equivalents and is used to compare the equivalent dose between two different types of radiation, whereas BED is used to compare effective dose between different fractionation schedules of the same radiation type.
8.2.9 Conformal Radiotherapy
The goal of conformal radiotherapy is to best adapt the high-dose field to the shape of the target using advanced computerized radiotherapy planning and delivery systems. Two important benefits emerged from conformal radiotherapy: the ability to reduce dose to normal structures near tumor and the ability to escalate dose to tumor, thus allowing for lower toxicity without compromising dose delivery to the intended target.
8.2.10 Stereotactic Radiation Therapy
SRT is highly conformal radiotherapy that involves the delivery of high doses of radiation in a single session or a few sessions through the use of a special coordinate system for target localization. SRS was originally developed to treat cranial lesions by using a stereotactic head frame to deliver a focal beam of external radiation. Nowadays SRS often refers to the use of SRT in a single session with the goal of delivering a highly focal and ablative dose of radiation with extreme accuracy and precision (typically 0–2-mm PTV margins) and steep dose gradients toward the critical OAR. With the advent of multifraction SRS, the term fractionated SRT (FSRT) is sometimes used to distinguish this from single-treatment SRS, although some use the acronym SRS to refer both to single fraction and to a few fractions. Stereotactic body radiation therapy (SBRT) or stereotactic ablative radiation therapy (SABR) are used when treating extracranial sites with FSRT. SBRT is often delivered in three to six sessions of doses > 6 Gy per session.
8.2.11 Image-Guided Radiation Therapy
IGRT, one of the more significant innovations in radiotherapy, is used together with conformal radiotherapy to reduce the PTV margin by minimizing the positional uncertainty of CTV and OAR targets. IGRT can provide high-resolution 2D, 3D, and 4D images to localize target position and capture motion and volume changes during each treatment, ensuring accurate placement of the radiation field according to the initial radiation treatment plan. IGRT is instrumental for SRT, which involves very small margins.
8.3 Principles of Radiation Physics and Radiobiology
Therapeutic radiation is ionizing radiation (IR), which consists of photons and charged particles. A key characteristic of IR is its capacity for releasing large amounts of local energy to break chemical bonds and produce a biologic effect. Electromagnetic radiation, such as photons, is indirectly ionizing radiation, whereas charged particles such as electrons, protons, and heavily charged ions are directly ionizing radiation.5
8.3.1 Electromagnetic Radiation
High-energy X-rays (photons) and gamma rays are the most common type of IR used for radiation therapy. Both photons and gamma rays are indirectly ionizing radiation. As they pass through biologic tissue, they deposit energy that produces electrons that then interact with the target. Photons and gamma rays have similar properties but differ in their origin and their ability to penetrate biologic tissue (Fig. 8.5). Photons are generated by linear accelerators (LINACs) that accelerate electrons at high energy toward a metal target (typically tungsten). The electrons lose kinetic energy when colliding with the metal target, and photons are produced as energy is conserved. Modern LINACs can produce photons that comprise a spectrum of energies expressed in megavolts (MV), where the number (e.g., 6 MV) refers to the maximum energy of the spectrum, which determines the depth of tissue penetration. Photons are the predominant type of radiation used today (e.g., intensity-modulated radiation therapy [IMRT]). Gamma rays are emitted by the nuclear decay of radioactive isotopes and can penetrate biologic tissue to a specific depth. For example, the Gamma Knife (Elekta) system uses cobalt-60 sources to generate gamma rays.

8.3.2 Particle Radiation
Unlike photons, charged particles such as protons, electrons, and other heavy charged ions directly interact with their biologic target. They are clinically useful in the treatment of skull base neoplasms because of their favorable depth-dose distribution characteristics (Fig. 8.6).6 Protons in particular, because of their relatively large mass, can penetrate tissue with less lateral side scatter than lighter particles such as electrons. This prevents broadening of the proton beam in tissue, so that less unintended dose is delivered to adjacent normal tissue.

Perhaps the most attractive feature of proton therapy is the Bragg peak (Fig. 8.7a), which represents the maximum range of the proton beam, such that few protons penetrate beyond this distance, even by a few millimeters. In clinical use, several energies are used to produce multiple Bragg peaks and thereby create a broader peak called an SOBP, which can be applied to cover the entire tumor (Fig. 8.7b).

Proton beam therapy is useful for skull base tumors such as clival chordomas, as a high dose can be delivered to the tumor, whereas critical structures such as spinal cord or brainstem distal to the proton beam receive minimal to no radiation. Newer developments include use of a spot scanning technique, which uses a pencil-thin proton beam (typically 5–10 mm wide). Treatment is delivered by scanning and superposition of a pencil-thin proton beam for increased precision and tailoring to the tumor shape. The availability of particle beam therapy is limited to specialized facilities. As of December 2019, 40 medical centers in the United States, and 65 centers in Asia and Europe offer proton beam therapy in accordance with the guidelines of the Particle Therapy Cooperative Group (PTCOG).
Carbon ions are heavier than protons and may bring additional biological advantages while improving dose distribution, owing to their high LET and RBE.7 Carbon ions are a potential breakthrough in radiotherapy, because their Bragg peak can reach even higher LET values than protons (close to those of alpha particles), producing high levels of irreparable DNA damage independent of free radical production, which is lethal to the cell. In carbon ion therapy, normal tissues are exposed to low-LET radiation similar to that associated with X-rays while the target receives high-LET radiation. As with proton therapy, carbon ion therapy requires expensive particle accelerators (i.e., cyclotrons or synchrotrons) and larger, more complex delivery systems than the more compact and less expensive LINACs (Fig. 8.8). Thus, particle radiation therapy, including IMPT and heavy ion therapy represent advanced and emerging technologies, albeit are of more limited access compared to photon-based therapies, within the field of radiation oncology aimed to improve cancer treatment.

As of December 2019, there are 13 heavy ion facilities in the world (six in Japan, four in Europe, and three in China). Although heavy ion therapy was developed in Berkeley, California, in the 1960s, the United States currently does not have a heavy ion center. Several multi-ion centers are also in operation, including: the Heidelberg Ion Therapy center in Germany, the Centro Nazionale di Adroterapia Oncologica in Italy, the Hyogo Ion Beam Medical Center in Japan, the MedAustron Wiener Neustadt in Austria, and the Heavy Ion Center in Shanghai, China. As of 2015, approximately 130,000 patients had been treated using proton therapy and nearly 20,000 with carbon ion therapy, according to the PTCOG website (https://www.ptcog.ch/archive/patient_statistics/Patientstatistics-updateDec2015.pdf).
8.3.3 Interaction with Ionizing Radiation
DNA and phospholipid membranes are widely considered to be the critical targets of ionizing radiation that produces a variety of observed biologic and molecular changes, including cell death, cell division delay, genomic instability, mutation, transformation, bystander effects, and adaptive responses (Fig. 8.9). The clinical manifestation can be tumor cell death or normal tissue toxicity. The type and severity of these effects depend on the energy and type of radiation, the composition of the target tissue, the cellular and molecular response, the duration of exposure, and the cellular microenvironment.

Photons and X-rays are considered sparsely ionizing (low-LET) radiation. Their primary mechanism of biological damage occurs indirectly, through production of highly reactive free radicals when the radiation interacts with molecules (mainly water) within the cell (indirect action). The most common interaction is with water molecules, producing shorted-lived yet extremely reactive hydroxyl radicals that can damage DNA through cell diffusion. This is the predominant mechanism of cell kill in low-LET radiation such as photons and gamma rays.5
Charged particles such as protons are densely ionizing (high-LET) radiation and cause cell damage by direct interaction with DNA and critical targets via the Coulomb force. This leads to ionization or excitation of atoms, causing a chain of physical and chemical effects that eventually produces biologic change that is the main mechanism of cell kill in high-LET radiation such as protons and carbon ions.5 Thus, for a given tissue type, densely ionizing radiation such as protons and carbon ions will have a higher RBE than sparsely ionizing photons and gamma rays.
8.3.4 Radiation Cell Killing
Cell death, from a radiobiology perspective, is the inability to reproduce indefinitely even if the cell maintains metabolic activity for a period. This phenomenon, which was recognized by radiation biologists for decades as “interphase death,” is characterized by an active and orderly process of death. A damaged cell may die at the first cell division after radiation or may undergo a limited number of cell divisions before death. This can be seen histologically when irradiated cells appear morphologically and metabolically intact but then undergo cell death after completing two to three cell divisions.
The mechanism of cell death (i.e., mitotic cell death, apoptosis, necrosis, or autophagy) depends on the radiation dose absorbed and the radiosensitivity of the radiated cell. Mitotic cell death, which occurs when a cell attempts to replicate, is an exponential function of radiation dose; it is considered the dominant mechanism of death, after standard fractionated doses. Radiation-induced apoptosis occurs less frequently and is most evident after lower radiation doses (typically 1.5–5 Gy).
8.3.5 Cell Survival Curve
Cell survival after a single dose of radiation is a probability function of the radiation dose in Gy absorbed. An absorbed dose of 2 Gy is large enough to cause hundreds of lesions in the DNA of an average cell. Most DNA lesions caused by 2 Gy radiation are repairable (sublethal damage). Sublethal damage can be repaired, often in hours, and does not lead to cell death. DNA lesions produced by densely ionizing radiation are usually not repairable or reversible (having suffered lethal damage) and eventually lead to cell death.
Cell survival after radiation exposure can be graphically expressed on a logarithmic curve by plotting the portion of surviving cells on the ordinate (y-axis) against radiation dose (in Gy) on a linear scale on the abscissa. A typical cell survival curve has a shoulder at the low-dose region followed by a logarithmic decline at the high-dose region (Fig. 8.10). The initial shoulder in the low-dose region is thought to represent sublethal damage (the amount of damage that the tumor cells must overcome with each additional fraction). The steeply sloped portion of the curve represents increased cell death as multiple instances of sublethal damage accumulate.

8.3.6 Linear Quadratic Model
The linear quadratic model is commonly used in radiobiology to explain the cell survival curve (Fig. 8.11). This model assumes that double-helix DNA is the critical target that results in cell kill, with double-strand breaks required for lethal damage. By contrast, a single-strand break in DNA is considered repairable, sublethal damage. A double-strand break can occur when a single track of radiation breaks both DNA strands or when two individual tracks create two individual single-strand breaks that are close to each other in space and time (Fig. 8.11).

The linear-quadratic model assumes that there are two components to cell killing. The linear (α) component is cell kill from a single event (i.e., one event causing a double strand break) and is a measure of the intrinsic radiosensitivity of the cell. It is proportionate to the dose (αD). The quadratic (β) component is cell kill from the interaction of sublethal events and represents the repairable portion of radiation damage. It is proportionate to the dose squared (βD2).
The linear-quadratic model is described by the formula
When radiation is fractionated, the linear-quadratic equation becomes
8.3.7 α/β Ratio
The linear quadratic model is used to determine the α/β of a given tissue (Fig. 8.12), which represents the relative contribution of α and β components needed to kill a cell using radiation. Each tissue type has its own α/β, so cell survival curves have different shapes for different tissues. It is the difference in α/β between normal tissues and tumor that allows for successful fractionation (Fig. 8.13).


8.3.8 Fractionation and the Four Rs of Radiobiology
In the early 20th century, fractionated radiotherapy replaced single-dose radiation as the major form of therapeutic radiation. The concept of fractionated radiotherapy is based on four well-established radiobiologic principles described by Rod Withers in 1975 as the four Rs of radiobiology: repair of sublethal damage, reassortment, repopulation, and reoxygenation. A fifth R, radiosensitivity, has since been added.8 The clinical effectiveness of fractionation was demonstrated in head and neck clinical trials developed during the 1970s and 1980s. These trials solidified 2 Gy per fraction per day as the gold standard for fraction size in the majority of malignant skull base tumors.
Fractionation exploits the radiosensitivity difference between rapidly growing tumor cells and most normal tissues (Fig. 8.4). The degree of normal tissue sparing from cancer cells depends on the dose per fraction, the type of tissue irradiated, and the type of radiation used. Hyperfractionation exploits differences in radiation sensitivity between tumor and normal parenchymal tissue to lower late toxicity risk in patients who receive re-irradiation. Accelerated fractionation addresses the risk of accelerated tumor repopulation that occurs after initiation of oncologic therapy. Combination of accelerated fractionation and hyperfractionation, once a common approach, resulted in improved tumor response but also increased acute skin and mucosal toxicity through its effects on the more rapidly proliferating epithelial cells of the skin and mucosa. Consequently, early re-irradiation trials for recurrent HNSCC used four cycles of 1.5 Gy twice daily for 5 days, with a protracted (9-day) treatment break between each cycle to mitigate severe acute toxicity concerns with re-irradiation.9
Repair of sublethal damage occurs when there is sufficient time for damage repair between fractions. Each time a radiation dose is fractionated, a shoulder reappears on the cell survival curve. The capacity to repair correlates with the size of the shoulder and is greater in mature, late-responding tissues than in rapidly proliferating tissues. The clinical implication is that decreasing the dose per fraction will increase sparing of late-responding tissues. This principle underlies hyperfractionation theory, which seeks to exploit the difference in repair between early- and late-responding tissues.
Reassortment studies have demonstrated that cells in the G2-M phase of the cell cycle are the most radiosensitive. In reality, cells of any given tissue can be found at various stages of the cell cycle. In an asynchronous population, radiation preferentially kills cells in the most sensitive phase of the cycle. The surviving cells will eventually reach the G2-M phase and consequently become more radiosensitive to the next dose. Reassortment occurs in tissues that have moderate to rapid cell turnover rate and is negligible in late-responding tissues. Fractionation exploits this phenomenon to decrease late-tissue complications and thereby improve the therapeutic ratio.
Repopulation occurs when the tumor cell population is depleted by radiation or cytotoxic treatment and a regenerative response of surviving clonogens (accelerated repopulation) is triggered. The timing and kinetics of regeneration vary by tissue type, with the magnitude depending on the number of remaining cells that retain their proliferative capacity. It is thought that tumor repopulation during radiation treatment breaks, after a subtotal resection surgery, or after chemotherapy accounts for the majority of treatment failures/tumor recurrences. Clinically, Rosenthal et al demonstrated that a total time from surgery to completion of radiotherapy (treatment package time) > 100 days is correlated with decreased local control in patients treated with surgery and adjuvant radiotherapy for head and neck squamous cell carcinoma (SCC).10 When the regenerative capacity of a tumor exceeds the critical acute-reacting normal tissue tolerance, therapeutic gains can come from shortening the overall course of radiotherapy, in what is called accelerated fractionation (see later this chapter).
Reoxygenation of the tumor microenvironment plays an important role in determining tumor radiosensitivity. In animal models, lack of reoxygenation is a major cause of tumor radiation resistance. Reoxygenation occurs when hypoxic cells closer to the center of the tumor become oxygenated again as a result of tumor shrinkage or reduced oxygen demand. This process is determined by a number of different mechanisms, including changes in interstitial pressure of micro vessels and cell proximity to the blood supply and the presence of certain radiosensitizing systemic agents.
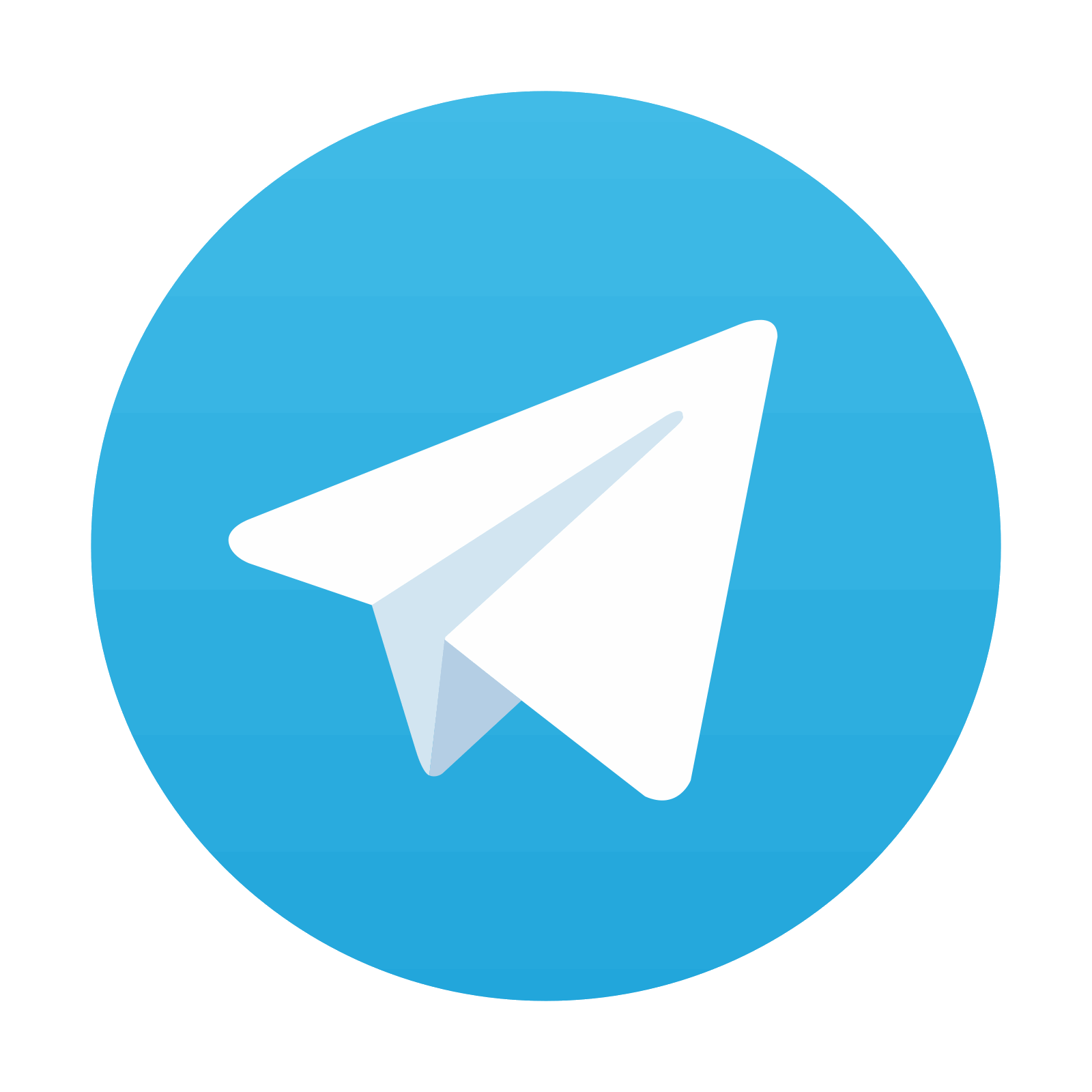
Stay updated, free articles. Join our Telegram channel
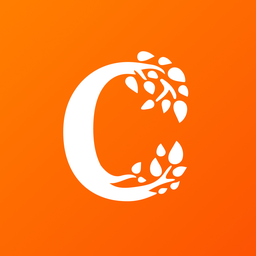
Full access? Get Clinical Tree
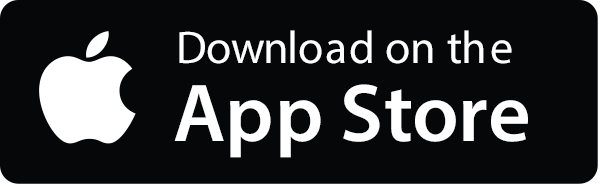
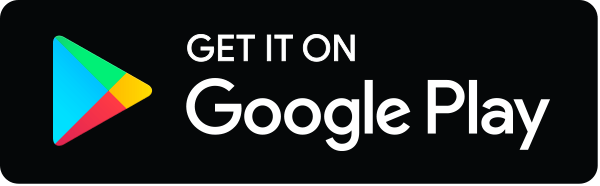
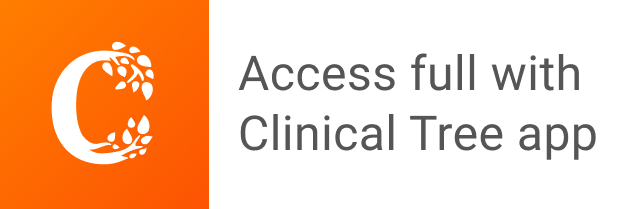