Figure 19.1 fMRI correlates of attention-based rehearsal of locations in working memory. The data illustrated here correspond to a delayed-location discrimination task in which subjects, while fixating centrally, judged whether a probe stimulus was closer to or further from central fixation than had been a target stimulus that had been presented 7 s previously. The delay period was unfilled. (a) illustrates the delay-evoked activity in a representative participant. Structural regions of interest (ROIs) are identified by translucent colors – superior parietal lobule (SPL; dark blue), intraparietal sulcus (IPL; red), premotor cortex (PMC; green), frontal eye fields (FEF; red), superior frontal cortex (SFC; orange), dorsolateral PFC (DLPFC; fuchsia), ventrolateral PFC (VLPFC; light blue) – and are overlaid by delay-responsive voxels, which appear yellow and orange. (b) illustrates trial-averaged fMRI data, by hemisphere, from the SPL delay-responsive voxels of the participant illustrated in (a). (c) illustrates quantitatively the delay effects (delay-epoch covariates scaled by their parameter estimates) estimated by the statistical analysis of the data illustrated in (b). (d) illustrates trial-averaged fMRI data, by hemisphere, from the IPS voxels illustrated in (a). (e) illustrates quantitatively the delay effects estimated by the statistical analysis of the data illustrated in (d). See also color plate 9. (f) illustrates the group results (mean and 95 per cent confidence interval, n = 9) from this study, which indicate that the contralateral bias (referred to on vertical axis as the ‘Laterality Index’) of delay-period activity was significantly different from 0 in Brodmann Areas (BA) 18 and 19 of the occipital cortex, and in IPS and SPL, but not in any of the frontal cortical ROIs. This contralateral bias is an operationalization of an attention-based rehearsal effect. For complete details, see Postle, Awh, Jonides et al. (2004). See also color plate 9.
the impending saccade (Curtis, Rao and D’Esposito 2004; Quintana and Fuster 1992; Takeda and Funahashi 2002, 2004) have found prospective motor coding-related activity in the caudate nucleus, the premotor cortex (including FEF), and dorsolateral PFC. Neurons of the dorsolateral PFC also register updates of the trial-ending saccade metrics when these are changed during the trial (Fukushima, Hasegawa and Miyashita 2004). These brain systems are likely involved in the sensory-motor coordinate transformation necessary for creating a prospective motor code, and/or in the STR of the motor plan itself.
Although attention-based rehearsal and prospective motor coding are summarized here as distinct mechanisms, each may simply represent a different point along a single sensorimotor continuum. (p.337) This would follow from evidence for close linkages between spatial attention and motor control (e.g., Andersen et al. 2004; Fuster 1995; Goldberg, Bisley, Powell et al. 2002; Goodale and Westwood 2004; Hamker 2005; Hoffman and Subramaniam 1995; Moore and Fallah 2004), and may be reflected in the trend of decreasing contralateral bias in delay-period activity (an index of attention-based rehearsal) that is seen as one proceeds rostrally from peristriate, through parietal, premotor, and finally prefrontal cortex (Figure 19.1). Theeuwes and colleagues (2005) have also noted the ‘evidence for a strong overlap between visual working memory, spatial attention, and the oculomotor system’, and use this to articulate an emergent-property view, suggesting that it is possible ‘that working memory is “nothing more” than the preparation to perform an action, whether it be oculomotor, manual, verbal, or otherwise’ (pp. 198–199).
Object identity
The literature on the neural bases of object working memory of the monkey point to an important role for advanced stations of the ventral (or ‘what’) visual object processing stream. As is the case with spatial delayed response, sustained delay-period activity is observed in several brain regions during object delay tasks, including in inferotemporal (IT) cortex (Miller, Erickson and Desimone 1996), anterior IT cortex (Nakamura and Kubota 1995), and entorhinal cortex (Suzuki, Miller and Desimone 1997) – all in the temporal lobe – and in PFC (e.g., Miller, Erickson and Desimone 1996; Rao, Rainer and Miller 1997; Wilson, O’scalaidhe and Goldman-Rakic 1993). Many of these temporal lobe regions, as well as PFC, demonstrate sustained object-specific delay-period activity that is not disrupted by intervening distracting stimuli. The necessity, for successful STR of object identity information, of the delay-period activity in two of these regions has been (p.338) investigated by Petrides (2000). This study demonstrated a double-dissociation of working memory functions attributable to PFC vs anterior IT cortex: lesions of PFC did not impair memory for the selection of one among two object stimuli across long (90 and 120 s) delay periods, but did disrupt memory for the selection of one from among a set of three, four, or five items across shorter (10 s) delays; whereas lesions of anterior IT cortex had the converse effect. These results are logically inconsistent with the idea that PFC is a necessary neural substrate for the STR of object information. Rather, they support an alternative view that the STR of information depends on anterior IT context (and, perhaps, entorhinal cortex), whereas control functions, such as the monitoring of multiple mnemonic representations and/or the selection from among them, are supported by PFC.
In the human, fMRI studies of n-back (Nystrom et al. 2000; Postle, Stern, Rosen et al. 2000) and delayed-recognition (Postle and D’Esposito 1999) working memory for location versus identity of abstract geometric shapes have each found shape-specific memory-related activity in ventral temporal and occipital cortex, but not in PFC (Figure 19.2).1 A subsequent fMRI study employed a multistep ABBA-like design intended to filter out delay-period activity that may be correlated with, but not necessary for, the STR of face identity. The task featured three 7 s delay periods that were interposed between the presentation of the first and second, second and third, and third and fourth stimuli. The logic was that the multiple distracting events in this task might serve to filter out activity from the first delay period that wasn’t involved directly in storage, because only voxels whose activity was necessary for retaining the memory trace to the end of the trial would be expected to maintain their activity across distracting stimuli. The hierarchical analysis procedure proceeded in three steps: First, Delay 1-sensitive voxels (presumed to represent the superset of the neural correlate of mnemonic representation of the target face) were identified; second, the Delay 1 voxels that remained active during Delay 2 were identified; and third, the voxels from Step 2 that retained their activity during Delay 3 were identified. As expected, the results from each subject revealed Delay 1-specific activity in many brain areas, including PFC, posterior fusiform gyrus of the temporal lobe and posterior parietal cortex. In each subject, however, only a subset of these voxels retained the Delay 1 signal during Delay 2, and posterior fusiform gyrus was the only region in which voxels retained the signal during Delay 3 in each subject (Figure 19.3, Postle, Druzgal and D’Esposito 2003). Other studies of working memory for faces are also consistent with an important role for posterior fusiform gyrus in the STR of face information (Druzgal and D’Esposito 2003; Ranganath, Cohen, Dam et al. 2004; Ranganath, DeGutis and D’Esposito 2004), and of analogous, nonfrontal, visual areas for other classes of visual stimuli (Ranganath, DeGutis and D’Esposito 2004; Todd and Marois 2004, 2005; Vogel and Machizawa 2004). Thus, working memory for the identity of objects is associated with sustained activity in the very brain systems that are responsible for the visual perception of these stimuli.
Verbal material
Within the domain of overtly language-based material, neuroimaging studies designed to identify the neural loci of working memory storage have, for the most part, pinpointed left posterior perisylvian areas (e.g., Awh et al. 1996; Hickock, Buchsbaum, Humphreys et al. 2003; Paulesu, Frith and Frackowiak 1993; Postle, Berger and D’Esposito 1999; Rypma and D’Esposito 1999) – areas (p.339)

Figure 19.2 Delay-period activity maps and time series plots from an fMRI study of a two-step delayed-recognition of location and shape task (‘what’-then-’where’ and ‘where’-then-‘what’ trials were randomized throughout each scanning session). (a) illustrates location (‘where’) and shape (‘what’) delay-period activity (circled) in the same slices through dorsolateral PFC of a representative subject. The dorsolateral PFC ROI is rendered in translucent white overlays. This figure illustrates the marked degree of overlap in dorsolateral PFC delay-period activity in the two conditions. (b) illustrates trial-averaged time series extracted from the voxels illustrated in (a). Values are plotted in arbitrary units of fMRI signal intensity; gray bars along the horizontal axis indicate the timing of the two delay periods during the trial. Note the similarity of fMRI signal intensity changes in spatial and object delay periods. (c) illustrates ‘where’ and ‘what’ delay-period activity in the same slices through ventrolateral PFC in a different representative subject. All display conventions for this and subsequent activation maps are the same as in (a). (d) illustrates trial-averaged time series extracted from the voxels illustrated in (c), with all display conventions for this and subsequent time series plots the same as in (b). (e) illustrates delay-period activity collapsed across ‘where’ and ‘what’ conditions from superior parietal lobule of a representative subject and (f) illustrates the trial-averaged time series plot from these voxels. Note that in contrast to (b) and (d), the delay-period activity is greater for ‘where’ than for ‘what’ in both trial types. (g) illustrates delay-period activity collapsed across ‘where’ and ‘what’ conditions from inferior temporal cortex of a representative subject and (h) illustrates the trial-averaged time series plot from these voxels, which is greater for ‘what’ than for ‘where’ delay periods in both trial types. (For complete details, see (Postle and D’Esposito, 1999).)

Figure 19.3 fMRI results from a multi-step delayed face-recognition task (behavioral task described in the text, and in Postle, Druzgal, and D’Esposito, 2003). The Delay 1 column illustrates the statistical maps identifying voxels in frontal cortex (FC) and fusiform gyrus (FG) with Delay 1-specific activity (critical voxels are circled). Delay 1 activity in FC was located in: right hemisphere middle frontal gyrus (MFG, Brodmann’s area [BA] 9/46) and inferior frontal gyrus (IFG, BA 44) in subject 1; left MFG (BA 8) in subject 2; left MFG (BA 9/46) in subject 3; right superior frontal sulcus (BA 8) in subject 4; and IFG (BA 44) and premotor cortex (BA 6) in subject 5. FG Delay 1 activity was located bilaterally in subjects 1, 3, 4 and 5, and in right hemisphere in subject 2. The Delay 2 column illustrates the statistical maps identifying voxels in FC and FG with Delay 2-specific activity within the search space comprising the voxels that had been identified in the Delay 1 column (translucent blue). The Delay 3 column illustrates the statistical maps identifying voxels in FC and FG with Delay 3-specific activity within the search space comprising the voxels that had been identified in the Delay 2 column (translucent blue). This Delay 3 activity can be interpreted as a candidate neural correlate of the STR of face information. Such activity, identified with arrows, was seen in FC only in subject 1 (right MFG [BA 9/46]), and in FG bilaterally in subjects 1 and 5, in right hemisphere in subjects 2 and 3, and in left hemisphere in subject 4. See also color plate 10
Concerns about the ‘activated LTM’ account of the STR of information for working memory, and possible resolution
The preceding sections of this chapter have summarized evidence that the STR of information in working memory is accomplished via sustained activity in anatomical networks whose principle function is not mnemonic. Variants of this idea, particularly that the STR of information in working memory is accomplished via the temporary activation of LTM representations (e.g., Anderson 1983; Cowan 1995; Oberauer 2002; Ruchkin, Grafman, Cameron et al. 2003) has met with considerable resistance from many quarters (see, for example, the commentary following Ruchkin, Grafman, Cameron and Berndt 2003). Some of the concerns are in the following vein: If working memory is accomplished via the temporary activation of long-term memory representations, how is the ordinal position of items in working memory retained? How can one account for the flexible transformation of information in working memory? and Working memory often represents the ‘here and now’ of a situation, and thus contains detail specific to the present moment that cannot be derived from the mere activation of representations in semantic or episodic memory. What follows is a consideration of how an emergent-processes view of working memory can addresses these concerns. To do so, however, requires first introducing a second principle of the emergent processes account, that of multiple encoding.
Multiple encoding, the idea that mnemonic representations can include a multiplicity of features, is a venerable idea in memory research (e.g., Estes 1955; Melton and Martin 1972). For example, Wickens (1972, 1973) demonstrated that words can be encoded, in parallel, according to their semantic attributes, to the physical characteristics of their presentation at the time of encoding, and to many ‘other’ attributes (such as language, frequency, representing symbol, and imageability). This idea has also been fundamental to the memory systems models of working memory, as seen, for example, in the recoding of visually presented alphabetic or numeric characters into a phonological code (Baddeley 1986). More recently, several studies have extended the principle of multiple (p.342) encoding to other domains of information. One example is that the working-memory representation of visually presented object stimuli may encode not only the visual features of the object (e.g., size, color, texture, shape), but also verbal information that is spontaneously associated with the visual stimulus by the subject (Postle, D’Esposito and Corkin 2005; Postle, Idzikowski, Della Salla et al. 2006; Simons 1996). In addition to recoding, working memory representations can also incorporate incidental encoding-related context. This is seen, for example, when running span (or ‘updating’) performance suffers when the grouping of stimuli at presentation is violated by the requirement to ‘drop’ some of a group’s items from the memory set but to keep others. This effect persists despite explicit instructions to subjects to ignore grouping information, despite intensive training and across different rates of stimulus presentation (Postle 2003). Other work has shown that the STR of letters is also sensitive to study-test manipulations of the color in which items are presented (despite instructions to ignore color), and to study-test changes in environmental context (Postle 2003; unpublished observations).
Now, back to the list of concerns laid out at the beginning of this section. The first had to do with maintenance of ordinal position of items represented in working memory. This is not a problem for the emergent-processes framework, because maintenance of ordinal position is a function for which the speech production system is well-suited (e.g., Bock and Levelt 1994). Thus, if the information in working memory is being represented, in part, in an articulatory code, (covertly) cycling this information through the speech production apparatus (e.g., Page and Norris 1998) would be a way to accomplish memory for order without resorting to a special-purpose memory system. (In such a case, one could invoke the operation of an ‘articulatory loop’, but to do so would seem to needlessly relabel a system that already exists.) The principle of multiple encoding permits the verbal recoding explanation to extend to working memory for all types of information, with the exception of egocentrically encoded location. Working memory for location is invariably insensitive to concurrent verbal interference (Logie 1995; Postle, Idzikowski, Della Salla et al. 2006). Consistent with this reasoning is the fact that memory for order is known to be superior when items can be represented with a verbal code (e.g., Glenberg and Fernandez 1988; O’Connor and Hermelin 1972, 1973). An analogous explanation can be invoked for ordinal memory for egocentrically encoded locations (as is required, e.g., by the Corsi blocks task) if one allows for contributions from the oculo-and/or skeletomotor system, because ordering and sequencing are also fundamental to the control of these systems.
What about the flexible transformation of information held in working memory? We know from the problem-solving literature that the ability to rerepresent information in a different format, or to consider it from a different perspective, can be important for solving problems. Similarly, the ability to represent an item (or a piece of information) in multiple codes, despite the unimodal channel by which it may have been perceived, should facilitate one’s ability to manipulate or transform the representation of this information.
Finally, what of the representation of the subjective present? The multiple encoding principle holds that, for example, when one is asked to remember the seven digits of a telephone number, there is more to this process than the retrieval into conscious awareness of the seven lexical representations that were a part of one’s knowledge base prior to being given the number to remember. Also represented in working memory can be, for example, information about who spoke the telephone number, about the timber, volume, and tone of the talker’s voice, about one’s affective classification of the talker, about whose telephone number it is, about other telephone numbers that are similar, about the ambient illumination in the room in which the number was spoken, and so on. This analysis makes clear that working memory can be construed as a special case of conscious awareness – conscious awareness of information that is not currently accessible in the environment.
(p.343) Shifting theoretical perspectives on nondeclarative memory: an analogy for working memory
The shift toward an emergent-properties view of working memory function has parallels in the recent evolution of our understanding of nondeclarative, or implicit, memory phenomena. Modern research in this area began with the characterization of the amnesic syndrome, and the fact that dense anterograde amnesia spared memory for many types of information, provided that this memory was probed in the right way. This included mirror-reversed visuomotor transformations (Milner 1962), routes through a tactually guided maze (Corkin 1965), line drawings (Milner, Corkin and Teuber 1968), and words (Warrington and Weiskrantz 1968). These and subsequent observations led to a taxonomic approach of cataloguing the many nondeclarative memory ‘systems’ that showed sparing despite anterograde amnesia, among these, abilities organized as skills, priming, dispositions andnonassociative(Squire 1987, 1992); structural-perceptual and lexical-semantic(aka ‘conceptual’) memory subsystems (Gabrieli et al. 1994; Keane, Gabrieli, Fennema et al. 1991); and visual word form, structural description, and auditory word form perceptual representation systems(Schacter 1990, 1994). This enterprise was particularly useful in delineating the boundaries of the kinds of memory performance that are dependent on the medial-temporal diencephalic anatomical system that supports declarative memory. As this work progressed, however, it also became apparent that an ever-subdividing taxonomy of nondeclarative memory systems and subsystems, seemingly growing with each new task and each new patient group tested, was becoming an increasingly unwieldy way to understand these empirical phenomena (e.g., Roediger 1990). Paralleling these theoretical concerns were developments in our understanding of the neurophysiological consequences of repetition. Most general was the fact that plasticity is a property of virtually all elements of the nervous system. More specifically, such plasticity could take the form of a signal increase or decrease, or in the expansion or contraction of a cortical representation, depending on the context in which repetition occurred.2Additionally, such plastic changes were invariably associated with changes (typically, improvements) in performance. Therefore, alternative accounts of nondeclarative memory began to emerge that emphasized the repetition-induced biasing of stimulus processing and/or task performance (e.g., Butler and Berry 2001; Postle and Corkin 1998, 1999). From this alternative perspective, nondeclarative memory didn’t reveal memory systems. Instead, it simply resulted from plasticity in the networks engaged by the task. In the past few years, invocation of nondeclarative memory systemsare increasingly infrequent, and have largely been replaced by studies that use repetition-related changes in physiological signals as probes with which to evaluate cognitive processes (e.g., Boynton 2004; Henson, Shallice, Gorno-Tempini et al. 2002; Stoeckel, Pollok, Schnitzler et al. 2004; Winston, Henson, Fine-Goulden et al. 2004; Yi and Chun 2005). By analogy to this evolution in viewpoints on nondecarlative memory, a similar change is now taking place with working memory.
The systems approach to working memory has, without question, been a remarkably fruitful one from which to launch the study of this cognitive phenomenon. This approach is becoming increasingly difficult to sustain, however, as our understanding of the cognitive and neural bases of working memory grows ever larger and more detailed. Particularly salient in this regard has (p.344) been the building evidence that the STR of information is supported by sustained activity in regions whose primary function is not working memory.
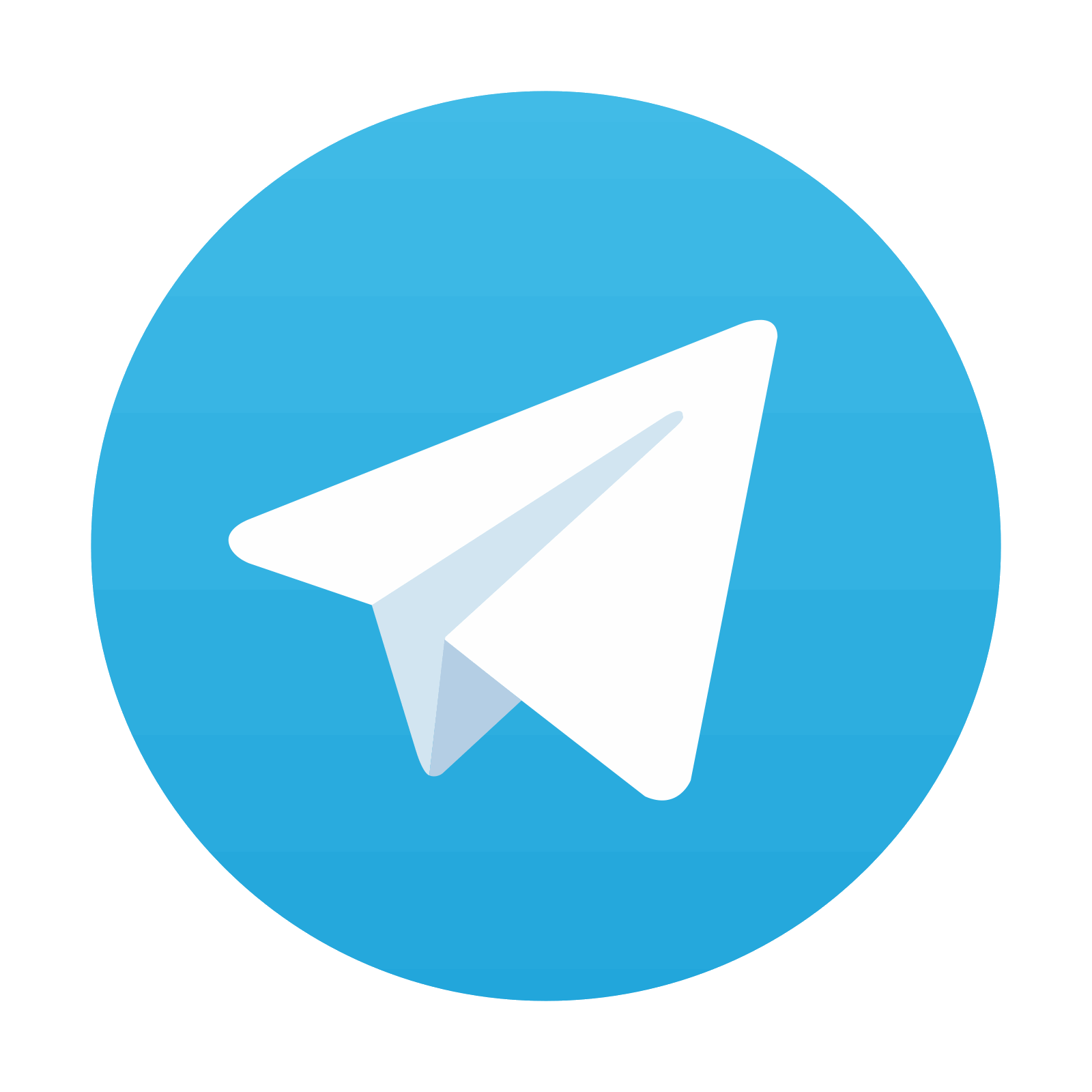
Stay updated, free articles. Join our Telegram channel
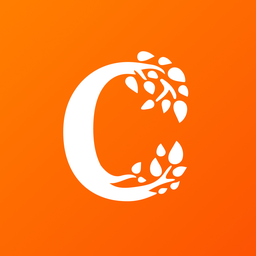
Full access? Get Clinical Tree
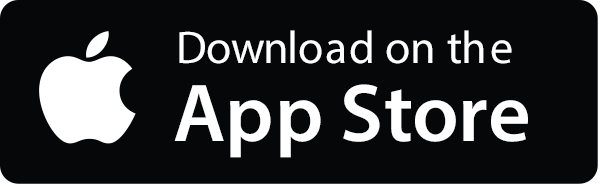
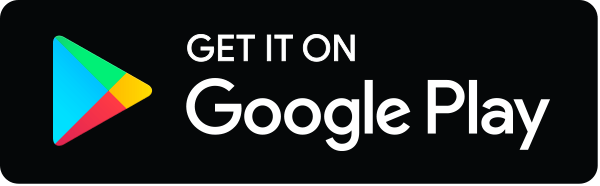