Chapter 10 Pulsed and continuous shortwave therapy
INTRODUCTION
Shortwave therapy (SWT) involves the coupling of high-frequency electromagnetic (EM) energy to the tissues to treat a wide range of musculoskeletal or neurological conditions. Shortwave generators utilize the non-ionizing radiation of the electromagnetic (EM) spectrum. The frequency range of 10-100MHz of EM constitutes the radiofrequency (RF) band that contains short, medium and long radio waves (see Fig. 2.20). The shortwave range of this band has been employed medically in the production of the physiotherapy-modality shortwave in its two forms: pulsed shortwave therapy (PSWT) and continuous shortwave therapy (CSWT). This chapter considers both modes of delivery and, where appropriate, differentiation between continuous and pulsed modes will be made. The term ‘diathermy’, although historically popular, will be largely omitted and the term ‘shortwave therapy (SWT)’ used in preference.
DEVELOPMENT OF SHORTWAVE THERAPY
Early experiences with high-frequency current started in the 1880s, when the French physiologist d’Arsonval passed a current of 1 ampere through his body and the body of his assistant. Despite the belief that a current of such strength would be deadly, they experienced only gentle warmth (Scott 2002). Based on this early work, experiments in the 1930s by the American physicist Arthur Milinowski and his colleague Dr Ginsberg focused on eliminating the heating effects and reducing the adverse outcomes associated with the application of CSWT (Lightwood 1989, Low & Reed 2000). Milinowski and Ginsberg introduced pauses in the electromagnetic field (EMF) output of the CSWT, which was thought at the time to allow for dissipation of heat and the prevention of thermal build-up (Arghiropol et al 1992). Their attempts culminated in 1936 in the production of an ultra-shortwave apparatus. They followed their efforts by experimenting on animals, but their task was terminated by the Second World War. In 1953, experiments were resumed and the first Diapulse apparatus (PSWT) was produced and marketed.
The revolution of Diapulse was followed by the production of Curapuls in 1970 and Megapulse in 1981, both of which were capable of producing continuous and pulsed output (Hayne 1984). Since then, the two modes of SWT have undergone fashionable shifts in utilization and, with the increasing move towards applying lower levels of energy (Watson 2000) and the belief that athermal modes of treatment could achieve therapeutic effects with minimal side effects, PSWT has become a more popular modality than CSWT (Al-Mandeel & Watson 2006, Shields 2003, Kerem & Yigiter 2002). Its increasing clinical use, however, has not been accompanied by an equivalent expansion of the supporting evidence. SWT remains an underexplored modality (Pope et al 1995) and relatively little is known of its bioeffects and its mechanism of action (Shields et al 2004), all of which warrant further research.
SHORTWAVE THERAPY GENERATORS
Therapeutic shortwave equipment is one of the many medical devices that utilizes high-frequency EMF. In an attempt to regulate the use of high-frequency currents in different disciplines, in 1947 the Federal Communication Commission assigned three frequencies at the short end of the RF band for the medical use of shortwave (Foley-Nolan 1990). These are: the frequency 40.68MHz (±620KHz) and a wavelength of 7.5m; the frequency of 13.56MHz (±66.25KHz) and a wavelength of 22m and the frequency of 27.12MHz (±6160KHz) and a wavelength of 11m (Prentice & Draper 2001). The majority of the research reported in this chapter relates to the use of the 27.12MHz frequency, which is the most widely employed in current clinical practice. The frequencies employed relate to international regulation rather than known optimal efficacy.
SWT generators produce high-frequency EMF by incorporating two electrical circuits: the machine and the patient circuits. The machine (or oscillator) circuit is composed of a high-frequency generator, amplifier (to raise the output to therapeutic levels), and a power supply (Fig. 10.1). The second circuit is the patient (or resonator) circuit, which is composed of a variable capacitor (to account for the changing capacity of the resonator circuit due to the type of tissue treated) and a method of transferring energy to the tissues; this is achieved by either capacitive or inductive electrodes.
The output of a SWT device can be delivered to the tissues in either a continuous or a pulsed mode. The difference between the two is that with CSWT the energy is delivered to the patient for the whole time of the treatment and, as such, it is primarily associated with thermal effects. With PSWT, the output is delivered to the tissues in a train of pulses of varying durations and repetition rate, as such allowing a high amplitude of energy to be delivered to the tissues with either thermal or non-thermal effects (Wadsworth & Chanmugam 1983, Watson 2006). Depending on the features of the machine being used, the main variables that can be controlled by the operator are pulse repetition rate (PRR), pulse duration (PD), pulse peak power (PP) and mean power (MP). In most machines MP can be controlled by changing PRR, PD and PP according to equation 10.1 (and illustrated in Fig. 10.2). Table 10.1 illustrates the typical ranges of pulse parameters available on modern pulsed shortwave therapy devices.
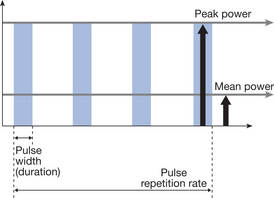
Figure 10.2 The essential relationship between pulse repetition rate, pulse duration, peak and mean power.
Table 10.1 Typical ranges of pulse parameters in modern pulsed shortwave therapy devices
Pulse parameter | Units | Typical range |
---|---|---|
Pulse repetition rate (frequency) | Pulses per second (pps) or Hz | 26-800 |
Pulse duration | Microseconds (μs) | 20-400 |
Pulse peak power | Watts | 150-200 |
Applied mean power | Watts | 0.1-30+ |
PHYSICAL CHARACTERISTICS
An electric (E) field is set up whenever an electrical charges moves; this field is characterized by both its direction and its magnitude. An electrically charged particle, such as an electron or proton, placed within this field will experience a force (F). E and F are related according to equation 10.2:
The interaction between the field and the tissues is affected by a macroscopic property of the tissue called the ‘complex permittivity’; this is related to the dielectric constant (Delpizzo & Joyner 1987). The dielectric constant represents the depolarization characteristics of a tissue and is primarily dependent upon the water content. Complex permittivity is also a function of the field frequency and, therefore, the propagation and attenuation of the electromagnetic waves are dependent upon frequency.
Although SWT units produce both an E and an H field in the tissue, their ratio will differ according to the mode of application, the type of electrode in use, the carrying frequency and manufacturing characteristics. Differences in the base (carrying) frequency of operation can also result in variance of the ratio of H and E fields (Hand 1990, Markov & Colbert 2000).
METHODS OF COUPLING SHORTWAVE THERAPY TO THE TISSUES
CAPACITIVE METHOD
Capacitor electrodes produce a higher proportion of E than H field in the tissues, with the field being stronger in the centre of the treated area (Prentice & Draper 2001). The strength of the field is governed by the electrode placement in relation to the tissue (there is a stronger field with the electrodes situated closer to the skin), the size of the electrodes (small electrodes have less penetration than medium and large electrodes) and spacing (uniform field in the tissues is achieved by using electrodes slightly larger than the treated area) (Hand 1990). The heat produced using capacitive method is the result of the movement of three components: charged molecules, dipolar molecules and non-polar molecules, and is governed by the strength of the E field and the conductivity of the tissues (more heat is produced in tissues with high conductivity), although capacitive application is actually expected to concentrate the field in the superficial tissues such as the skin and fat layers rather than the deep tissues such as muscles (Van der Esch & Hoogland 1991, Ward 1980). This is mainly caused by the reduction of field intensity as it propagates in the tissues. The refraction of the lines of force as they cross the muscle fat layer causes the loss of part of the applied field strength and termination of some field lines (Ward 1980). As the heating pattern with these electrode is mostly in the skin and subcutaneous fat layer, this method is possibly best suited for treating ribs, spine and areas of low subcutaneous fat such as hands and feet (Prentice & Draper 2001), and the use of capacitive delivery of SWT has diminished in recent years.
Two types of electrode are used with the capacitive method: air space plates and pad electrodes (Wadsworth & Chanmugam 1983).
Electrode arrangement
With conductive techniques, electrodes can be positioned in a contraplanar, coplanar, longitudinal or crossfire arrangement. In contraplanar, the electrodes are placed opposite to each other on either side of the treated area. The distance between the skin and the electrodes can be symmetrical if an even field is desirable, or the electrodes can be positioned with uneven distances if the aim is to concentrate the field on one side of the treated area (Scott 2002, Wadsworth & Chanmugam 1983). To achieve heterogenous heating, it is considered important that the centre of the electrodes is centred with the centre of the treated area (Garret 2000, He et al 2005). Figure 10.5 illustrates a contraplanar air-spaced electrode arrangement at the knee.
Electrodes can also be positioned in a coplanar arrangement (Fig. 10.6), where both electrodes are placed on the same aspect of the treated area. For safety reasons, the distance between the two electrodes needs to be more than the sum of skin electrode distance in order to result in better field distribution. Although this technique produces a more superficial field, the depth of the field can be increased by increasing the distance between the two electrodes (Martin et al 1991).
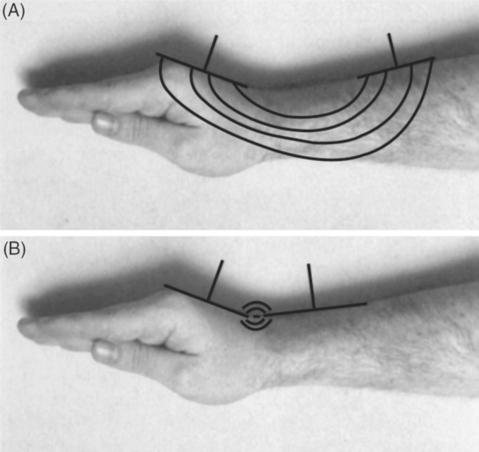
Figure 10.6 Coplanar electrode placement illustrating correct (A) and incorrect (B) electrode spacing.
Other electrode arrangements include the longitudinal application, when the electrodes are placed at either end of a limb parallel to the alignment of the tissues and the crossfire technique, when the electrodes are placed diagonally over the treated tissue for half the time and are then changed to the other diagonal for the rest of the time. Historically, this latter technique was used to treat cavities containing air, such as sinuses and the uterus (Forster & Palastanga 1985).
Electrode size
The size of the applicators is considered an important factor in determining the strength of the field in the tissues. The use of two electrodes of similar size results in uniform distribution of the field in the tissues. Using electrodes of different sizes, however, leads to the accumulation of the field on the side of the smaller electrode (Tzima & Martin 1994). Hand (1990) notes that applicators act as antennae for coupling the power to the tissues. When small applicators are used (small in relation to the other electrode or in relation to the size of the treated area) they act as poor radiators, decreasing the intensity of the field as the distance increases. This is believed to cause excessive heating at the superficial layers and reduction in the depth of penetration (Hand 1990).
INDUCTIVE METHOD
Inductive SWT can be applied to the tissues by means of either a drum or a cable electrode (Prentice & Draper 2001, Watson 2006). The inductive method produces predominantly an H field via a cable that is either wrapped around the extremity or coiled inside an electrode housing. The use of the monode or drum applicator has become the clinical norm in current practice, and the cable application is rarely employed. Unlike the conductive technique, the patient is not part of the circuit and the electrodes are placed perpendicular to the part to be treated (monode, circuplode) or wrapped around it (cable) (Hand 1990). A modern monode applicator is illustrated in Figure 10.7. Generation of the alternating high-frequency current in the cable produces an H field in the tissues, which is set up at right-angles to the direction of current flow (Scott 2002). The amount of E field emission from the monode-type electrodes is usually limited by using a Faradic screen. The strength of the H field is determined by the rate at which the current alternates and by the number of coils of the conducting wire contained within a conductor (Hand 1990).
The generated H field induces a secondary current in the tissues known as an eddy current (which consists of small circular E fields). Heat is generated as a result of the friction between eddy currents and intermolecular vibration of the tissue. It is believed that the effect of the H field produced by the inductive technique acts as a carrier for the eddy current, which acts as the main element responsible for the physiological effect gained during the application (Scott 2002). This form of heating is not associated with strong sensory stimulation as there is less superficial (skin and fat) heating and, as such, the heat may not be as obvious to the patient as in the capacitive application (Prentice & Draper 2001).
The inductive application is believed to result in selective energy absorption and heating. Tissues with a high electrolyte content and low impedance, such as muscle and blood, will be heated to a greater extent with the superficial layers such as skin and fat being minimally affected (Lehmann & DeLateur 1990, Watson 2006). Ward (1980) argues that with an inductive application there will be both superficial and deep heating. Structures such as blood and muscles will be heated as they have high dielectric content; however, the superficial fat layer will also be heated because fat is an inhomogeneous structure and usually incorporates areas of high conductivity found in the small blood and lymphatic vessels. These tissues lack an efficient way of dissipating heat and, as such, tend to absorb heat and concentrate it in the small blood vessels. Hand (1990) also argues that when the separation between the electrode and tissue is less than 3cm, high intensities of the power are absorbed by the fat layer. However, by increasing this distance the field is expected to penetrate up to a depth of 4cm. Although this study is one of the few that discussed the exact distance between inductive electrode and the tissues, it does not clearly state whether these findings were based on experimentation or on a theoretical model. Draper et al (1999) were able to demonstrate an increase in intramuscular temperature of up to 4°C using inductive electrodes. The inductive application (drum or cable method) can be employed with continuous or pulsed shortwave modes, although the most common combination in current practice is to use the drum applicator with shortwave in pulsed mode.
Drum electrode
The drum is composed of one or more monoplanar coils (sometimes referred to as a ‘pancake’ arrangement) encased inside a plastic housing (see Fig. 10.7). The applicator has the advantage of being very straightforward to set up, although the disadvantage with this technique is that the drum electrode is less compliant with the skin contour. Maximum penetration with this technique is believed to be 3cm, given that the subcutaneous fat layer does not exceed 2cm. This is believed to be caused by the distortion of the field when it passes the fat layer and crosses the fat-muscle interface, which is expected to lead to unwanted increase in temperature in the fat layer (Prentice & Draper 2001, Low & Reed 2000, Ward 1980). Examining seven types of applicators, Lehmann et al (1983) demonstrated (using human tissue substitute substances) that the specific absorption rate (SAR) ratio of muscle to fat heating with inductive electrodes could vary between 0.4 and 2.7:1.
Cable electrode
The cable is a thick, insulated wire with plugs on either end. It can be wrapped in a pancake arrangement and placed over the treated area or it can be wrapped around the extremity. It has the advantage of fitting the contour of the body, unlike air-space or drum electrodes. A distance of at least 1cm should be kept between the skin and the electrode, and a distance of about 5cm should be kept between the turns of the cable to prevent overheating (Prentice & Draper 2001). The cable application method is rarely employed in current clinical practice, the drum or monode applicator being considered more efficient to set up and thus more effective.
HEAT PRODUCTION WITH SHORTWAVE THERAPY
Shortwave therapy can be employed in either thermal or ‘non-thermal’ modes: the use of continuous SWT is always considered to be thermal, whereas pulsed SWT can bring about thermal or non-thermal effects. The rise in tissue temperature during the application of SWT depends on the SAR, which is the rate at which energy is absorbed by a known mass of tissue and is calculated in units of watts per kilogram (W/kg). SAR is a function of tissue conductivity and the electrical field magnitude. Tissue conductivity reflects the ease with which an electric field can be set up in the tissue. The SAR, and therefore the heating produced by SWT, is dependent upon the electrical properties of tissue within the electromagnetic field (Kloth & Ziskin 1996).
Electric field energy will concentrate in tissues with the greatest conductivity. It is accepted that tissues with high dielectric content, such as muscle and blood, are also good conductors of the current as they have the ability to absorb more energy and dissipate the resulting heat more efficiently (Scott 2002). Fatty tissues, on the other hand, have low dielectric constant and low conductivity (Ward 1980) and also tend to heat up, albeit for different reasons. Some have explained this by poor vascularity and the lack of thermoregulatory mechanism in the fatty layer (Wadsworth & Chanmugam 1983), whereas others suggest that it is the small blood vessels spread throughout the fatty layer that are responsible for retaining heat and the build-up of temperatures (Ward 1980). These views remain largely theoretical, with little directly supportive evidence.
It is accepted that CSWT application is always associated with heat production in the tissues unless applied at exceptionally low power levels. Pulsed-mode shortwave can be employed in a ‘thermal’ or ‘non-thermal’ fashion with the applied mean power (MP) being the main determinant. The thermal mode of PSWT can be achieved by using longer PD and high PRR. The non-thermal mode is achieved when short pulses are interposed by long interpulse periods. This is not strictly a ‘non-thermal’ mode, but that there is no net increase in temperature (ΔT) as the heat gained during the ‘on phase’ is dissipated by the circulating blood during the interpulse period (Scott 2002, Watson 2006). When long-duration treatments are applied with these parameters, it is possible that an accumulative heating effect may still be achieved. The differences between thermal and non-thermal effects of therapy are further considered in Chapter 8.
Heat generated in the tissues is the product of tissue resistance and current density as explained in equation 10.3 (Prentice & Draper 2001):
The second type of molecule, e.g. water, some proteins and hormones, possesses permanent electric dipoles. Normally, these dipoles are randomly arranged. Under the influence of an E field, these dipolar molecules undergo polarization and align themselves to the opposite charged pole of the E field. The alternating nature of the field causes the dipoles to rotate and collide, and the friction between these dipoles generates heat. The extent of this alignment is determined by the strength of the field (Hand 1990). Additionally, each of these polar molecules possesses a weak field of its own, extending from the positive to the negative pole and, when the substance is under the influence of the E field, the net result of these fields governs the electric properties of the matter. Dipolar molecules produce a mixture of real and displacement currents. Real current refers to the current that develops in the tissues and determines the electrical properties and heat production in a matter (Ward 1980) as opposed to the displacement current, which does not play a great role in the electrical properties of a matter (Scott 2002).
The third type of molecule is the non-charged molecule. The E field affects non-charged molecules by polarizing and distorting their electron cloud fields. Movement of the non-charged molecules in response to the E field results in displacement currents and as such contributes the least to heat production in the tissues, unlike the movement of charged molecules, which could result in real current. This is because the induced dipoles are not as strong as natural dipoles and tend to lose their properties as soon as the E field is removed (Delpizzo & Joyner 1987, Durney & Christensen 2000, Ward 1980).
Adey (1988) argues that EM energy absorbed by the tissues will always result in internal thermal energy regardless of the mode of application. The energy delivered to the tissues increases the kinetic energy of the molecules, resulting in random ionic movement. Atoms become excited and move into higher levels of energy, releasing photons of EMF, which may be transferred to other atoms or changed into heat (Low & Reed 2000, Scott 2002). Although no ΔT is detected externally on the skin, non-uniform heating occurs and microthermal effects are still occurring at the cellular level. However, these effects are believed to be caused by E rather than H fields (Durney & Christensen 2000).
Some argue that tissues could be irradiated with PSWT and that collision and friction of molecules could still be occurring; however, the proportion of E current converted into heat would be very slight and no real current is initiated in the tissues (Ward 1980), resulting in thermal agitation (Foster 1997), which lasts for the time of the ‘pulse on’ period (Michaelson & Elson 1996, Tenforde 1996). It could be added that even with tissues containing high dielectric molecules, under the influence of the E field it is the extent of dipolar alignment to the E field that determines the amount of heat produced, and this is dependent on the strength of the applied current and the amount of E field absorbed by these molecules (Hand 1990), which is governed by the proportion of displacement to real current that determines whether a given application is thermal or not. Longer treatment durations are expected to result in more energy delivered to the tissues, higher current densities, and a possibility of heat build-up compared to applications with short durations. It has not been customary so far to describe PSWT treatment doses in terms of power concentration (W/cm2), although it has been suggested (as with ultrasound) that this may in fact be the most appropriate term.
The changes in the field distribution in response to implanted metal in the tissues has been considered in detail by Virtanen et al (2006) and the clinical use of PSWT with metal implants is discussed by Seiger and Draper (2006).
MECHANISMS OF ACTION
It is believed that the response of biological systems to EM energy could be the result of either thermal or ‘athermal’ effects. The heat developing in the tissues after 20 minutes of application of SWT was found to peak at 15 minutes, after which it levelled off for 5 minutes and then started to decline slowly at a rate of around 1° per 5 minutes (Draper et al 1999, Valtonen et al 1973). Therapeutically, a temperature increase of more than 1°C is useful for mild inflammation and an increase between 2 and 3°C is helpful in reducing pain and muscle spasm, whereas an increase of 3-4°C is necessary to cause changes in tissue extensibility (Lehmann 1990, Prentice & Draper 2001). The demonstrated changes in cell behaviour were found to be reversible immediately on termination of application if the increase in temperature was less than 1°C (Michaelson & Elson 1996, Tenforde 1996).
The mechanism of response to EM energy is thought to occur at several sites within the cell. One of the main sites for interaction between the applied energy and biological tissues is thought to be the cell membrane. It is suggested that EM energy changes the rate of opening and the formation of ion channels across the protein bilayer found in the cell membrane (Cleary 1997). This changes the charged ion build-up at the surface and the way new molecules are bound to the surface (Polk & Postow 1996). Cations such as Na+ and K+ leak from inside the cell to the extracellular fluid under the influence of the energy (Cleary 1997), altering the intracellular and extracellular environments (Adey 1988). It is also expected that these changes might restore ion, oxygen and nutrient concentrations to a more balanced state (Markov & Colbert 2000). It is believed that the E field could change membrane selectivity to ions hence altering ion transport across the membrane (Hand 1990).
Whereas the response of the nucleus to exogenous EM energy is governed by the presence of ions such as Ca2+ (Low & Reed 2000), the mitochondria are thought to change cell function by altering the rate of cell metabolism (Cleary 1997). Pope et al (1989) demonstrated that the interior of the cell reacts to the applied current by reducing mitochondrion size, increasing endoplasmic reticulum size, decreasing the cell lipid size, migration of lipid to cell poles and an increase in activity of ATPase. The size of the response was seen to correlate with the amount of energy delivered to the cell. For example, the reaction was higher with PD 400ms, PRR 400pps, intensity 1, for 10 minutes than with PD 400ms, PRR 80pps, intensity 4, duration 10 minutes.
Microtubules are dipoles and are expected to respond to the alternating E field by rotating and colliding, thereby producing heat (Charman 1990).
All the above changes are expected to revert the electrical potential of the diseased cell, correct the endogenous abnormalities and restore normal cell function (Chapter 3; Nordenstorm 1983). SWT coupling to endogenous fields is believed to alter physiological processes (Ward 1980).
It is thought that the vasodilatation observed with SWT occurs as a result of the accumulation of waste products or the direct stimulation of smooth muscles of the vessels in response to heat (Ward 1980). Blood vessels are also thought to dilate in response to the stimulation of sensory nerve endings at the skin surface as a result of heat production, initiating an axon reflex (Kitchen 2002). With vasodilatation, there may be a decrease in blood viscosity to ease the flow of blood.
Increase in blood flow is proportional to the gradual increase of energy, as demonstrated by Erdmann (1960), who examined the effect of radiating the epigastrium on the blood flow in the feet of 20 adults. Erdmann recorded a noticeable increase in blood flow, which started to increase in the first 8 minutes and reached a plateau within 35 minutes of application. Blood flow readings returned to baseline by 30 minutes post treatment. The increase in temperature was 2°C in the foot and ranged between 0.5 and 1.5°C under the treatment head. However, this increase was not associated with change in core temperature. These results were further supported by Morrissey (1966).
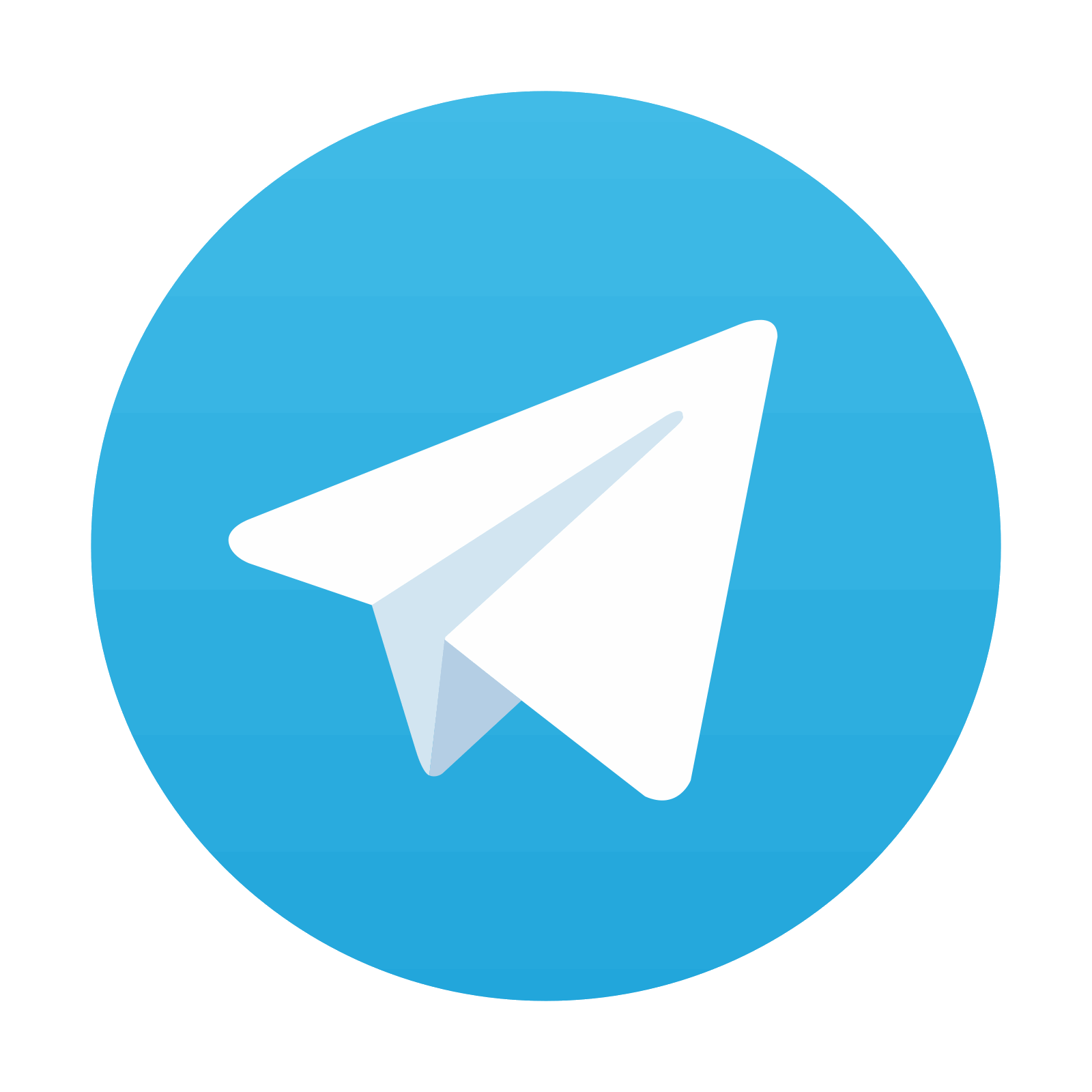
Stay updated, free articles. Join our Telegram channel
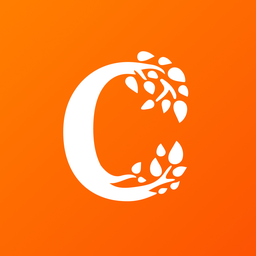
Full access? Get Clinical Tree
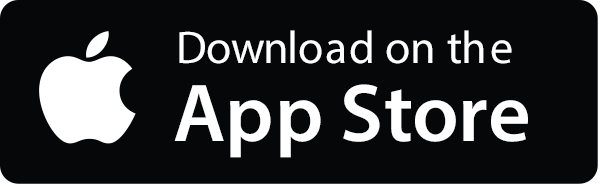
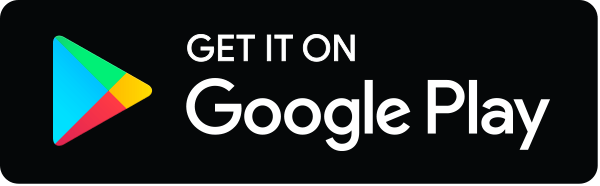