Fig. 1
CT scanner (Courtesy of General Electric, Inc.)
Magnetic Resonance Imaging
MRI provides visualization of structures that is very similar to those seen in autopsy studies. Figure 2 shows an example of an MRI. The MRI is a large magnet with varying field strength units depending on the particular magnet. Most clinical and research scans are at the 1.5–3.0 Tesla level. The Tesla level is a measure of field strength and indicates how fast the scanner is able to obtain a picture with good resolution. The process behind MRI consists of very complicated physics. In extremely simplified terms, hydrogen photons are numerous in the brain and these photons will align in the same direction as a magnetic field. MRI emits a radio-frequency pulse to deflect these protons at a predetermined angle to provide the best picture of a structure. When the pulse stops, the photons return to their original position. Altering the rate, duration, and intensity of these pulses provides a different type of visualization. An MRI is a series of these sequences and averages approximately 7–10 min to complete. Most research requires several sequences and takes around 45 min to 1 h to complete. Figure 3 provides an example of an MRI picture.
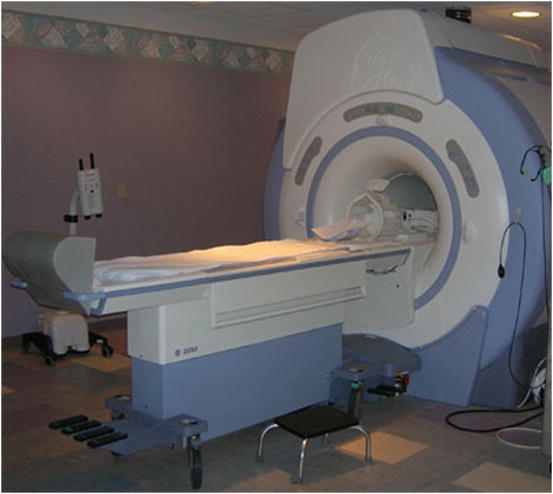
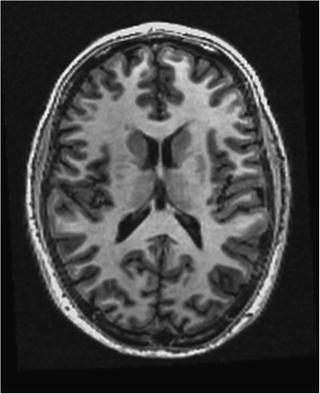
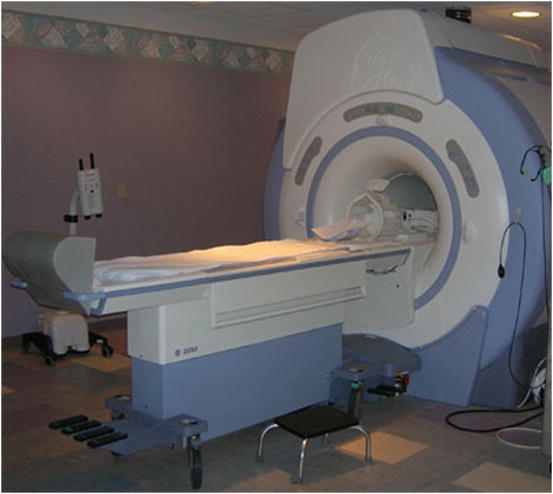
Fig. 2
MRI scanner
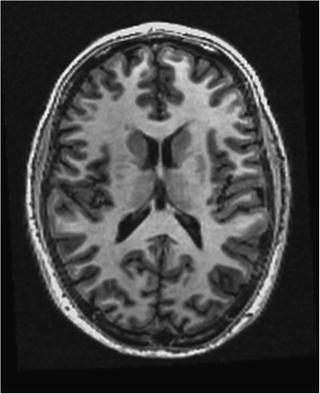
Fig. 3
Example of an anatomical and structural MRI
There are two different types of MRI sequences that are used clinically. Each is used for different purposes. The T1-weighted scan shows excellent anatomical detail of white and gray matter. Figure 3 illustrates the detail that can be obtained. T2-weighted sequences are sensitive to water content and are generally used to determine the extent of a lesion or a tumor. When a tumor is suspected, the MRI scan is good at isolating the tumor from surrounding swollen tissue (Filipek, Kennedy, & Caviness, 1992). While an MRI doesn’t use radiation, it is fairly expensive and generally used for children or for disorders that do not readily show on CT (i.e., lesions from multiple sclerosis).
Functional MRI. fMRI is a relatively new technique that allows for the visualization of brain activity by tracking cerebral blood flow deoxygenation or the blood oxygen level dependent (BOLD) signal. This signal is assumed to correlate with increased metabolic activity that is present when the brain is actively working on a problem or responding to stimuli. The oxygen level in the blood changes as the brain activity changes with oxygen being expended with use. Differences in oxygen levels in the blood create the BOLD signal and in turn affect the magnetic properties of the oxygenated and deoxygenated blood allowing for imaging (Luna & Sweeney, 2004). Figure 4 shows a map of functionality of a child watching a video.
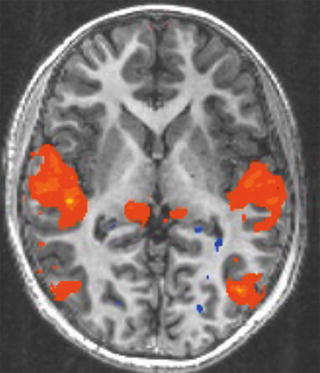
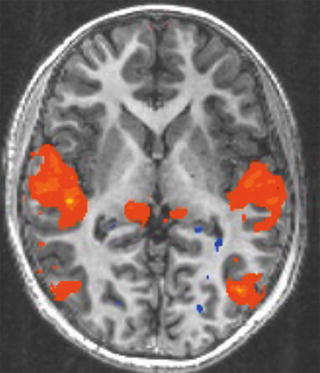
Fig. 4
Example of an fMRI map
Diffusion Tensor Imaging. Another more recent neuroimaging technique is diffusion tensor imaging (DTI). DTI allows for visualization of the white matter tracts in the brain and is a static picture. The DTI process is an analysis of the amount of perfused water in the long axons of the brain. As such, DTI can show orientation and density of white matter in the brain as well as provide the ability to analyze the axon fiber tracks. Figure 5 is an example of a DTI scan.
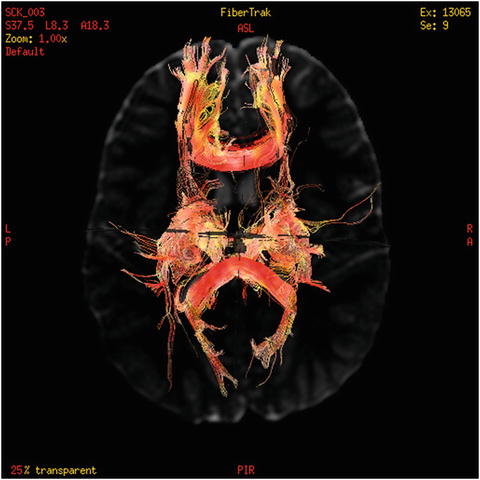
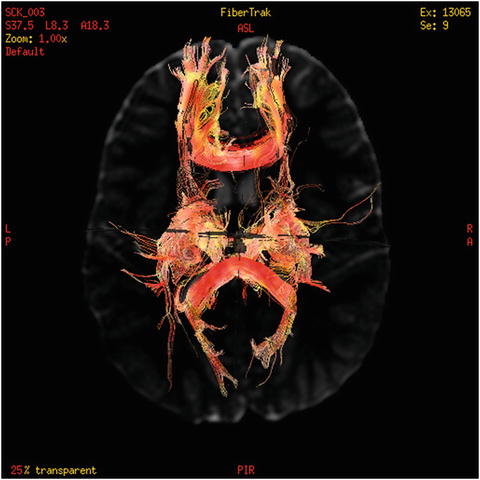
Fig. 5
Example of DTI
Methodological Issues in MRI Research
While neuroimaging is an exciting tool, it is also relatively new, and has some methodological issues that can make interpretation of the findings challenging. One issue, which is not unique to neuroimaging, is the issue of how the sample is defined. Age is a variable that can affect the findings, and in many early studies ages can range from 4 to 16, or even to 80 years of age, without attention to the differences that may be present. As seen later in this chapter, there are many structures that change with age, with some growing and others decreasing in volume (Castellanos et al., 1996). In addition, many studies contained mixed samples of gender and ethnicity making it difficult to understand whether there are gender or ethnicity differences among participants (DeCarli et al., 2005). Differences in co-morbidity, age, gender, and medication status have all been found to impact the findings and need to be recognized when interpreting a study. For instance, medication effects have been found to be related to structural differences in children with Attention Deficit/Hyperactivity Disorder (ADHD) (Semrud-Clikeman & Pliszka, 2006). Other aspects that have not been well controlled in many MRI studies are handedness (people who are left-handed may have speech/language lateralized differently than those who are right-handed), family history of a previous disorder (there may be genetically based differences), and true normalcy (functioning may be normal but the brain may be different).
MRI technology can also differ from study to study. Magnets vary not only in strength but also in acquisition time and in movement artifacts. Children in particular have difficulty remaining still within a closed space and those with developmental disorders experience even more difficulty. In many cases 20–40 % of scans may be discarded due to movement artifacts. It is likely that the most severe cases are either unable to cope with the scanning protocol or move so much that their scans are unusable. If this is the case, then the findings that are published may relate solely to milder cases and may not be informative of more severe types of disorders, especially with children.
Finally, an issue that is not often considered is that of power. Since there can be over 128 slices for each brain, and there are over a hundred brain structures that can be studied, it becomes obvious that statistical power cannot be sufficient for all of these comparisons. Without a data driven hypothesis for a study, the findings may be spurious due to Type II errors. At times neuroimaging research has been faulted for being atheoretical, or a new type of phrenology. Good research approaches the question by asking what do we believe underlies this difficulty/variable and how do our findings relate to theory? These questions are particularly germane to MRI research because many of the studies use very small samples—sometimes less than ten participants per group.
Brain structural differences between genders are just beginning to be explored. There is more information concerning the male brain than the female brain. Emerging evidence suggests that there are differences in selected structures including the corpus callosum, gray matter, amygdala, parietal lobe, and hippocampus (Semrud-Clikeman & Fine, 2009; Semrud-Clikeman, Fine, Bledsoe, & Zhu, 2012). With the aforementioned cautions in mind, the following section will review differences in brain structure throughout the life span.
Gender Differences in Childhood
The first 3 years of life is a crucial period of time for brain development. Functional and structural development is generally around 90 % complete by age 3, particularly for sulcal and gyral formations and size (Armstrong, Schleicher, Omran, Curtis, & Zilles, 1995; Caviness, Kennedy, Bates, & Makris, 1996; Giedd et al., 2004). There are several million neurons too many during this developmental phase and excess neurons are pruned back to make the brain more efficient. Additional environmental experiences and interactions create an elaboration of neuronal connections and increase the communication within and between neuronal networks (Huttenlocher, 1990). Myelination continues throughout childhood and into young adulthood (Jernigan, Trauner, Hesselink, & Tallal, 1991; Pfefferbaum et al., 1994; Wang & Young, 2014). Myelination is the formation of a white, fatty sheath that covers the axon and speeds the neuronal impulses. Neither myelination nor synaptic pruning significantly affect overall brain anatomy, thus, brain weight does not change during adolescence and young adulthood (Luna & Sweeney, 2004). In adolescence as well as in young adulthood, myelination increases in the frontal lobes and contributes to the eventual improved insight into behavior and the ability to plan and evaluate behavioral plans, aims, and long-term goals.
Age-related cortical and subcortical development has been found in total brain volume and white matter volume with decreases in gray matter during childhood and adolescence. The white matter increases were due to increases in volume in the frontal lobe and anterior cingulate in adolescents. In contrast the caudate and thalamus decreased in volume within this same time period for males but not females (Giedd et al., 1996). Males showed larger putamen and globus palladi regions compared to females; these areas are responsible for input of motor information. In general, male brains are 7–10 % larger than female brains (Giedd et al., 1996; Giedd, Castellanos, Rajapakse, Vaituzis, & Rapoport, 1997; Reiss, Abrams, Singer, Ross, & Denckla, 1996; Sowell, Trauner, Gamst, & Jernigan, 2002). However, with brain size factored in, females showed larger volumes in the temporal cortex and deep brain structures compared to males, while males had a larger cerebellum (8 % larger in males). The ratio of cerebro-spinal fluid (CSF) to brain volume also changes with age, with young children showing 2 % of total brain volume due to CSF and older children showing 4 % (Sowell et al., 2002).
Increases are also found for the amygdala and hippocampus for both genders during development (Giedd et al., 1997). There were differential changes with the amygdala increasing more significantly in volume for males while the hippocampus increased in volume for females. Some hypothesize that these differences are related to hormonal gender differences. Androgen (a male hormone) is more present in the amygdala (Clark, Maclusky, & Goldman-Rakic, 1988), while the hippocampus has more estrogen receptors (Sholl & Kim, 1989). These differences have been related to a theory that the X chromosome may be connected to volumetric differences in the caudate, thalamus, and gray matter of the cerebral cortex (Murphy et al., 1993), while others speculate that hormonal effects are related not just to specific brain structures, but to overall brain size and asymmetry of the hemispheres (Kelley, 1993). These hypotheses require further study.
To more fully study possible hormonal effects on brain development, Rose et al. (2004) evaluated children with congenital disorders associated with hormonal disturbance. Sixteen boys with congenital adrenal hyperplasia (CAH), which results in fewer androgens, completed an MRI. These boys were compared to 20 children with Klinefelter’s syndrome (XXY). Each clinical group was matched to controls by age. Findings were of smaller amygdalar volumes in the boys with either CAH or XXY. The boys in the XXY group showed smaller hippocampi volumes. A major limitation of these findings was that there were no behavioral or neuropsychological findings with which to compare the neuroanomalous results. Such data would have provided an opportunity to determine whether these structural differences related at all to the child’s ability to manage stressful situations. At this point it is only clear that there are differences in structures in these children with anomalous hormonal distribution.
Another structure which has shown to differ between the genders is the corpus callosum (CC) (Giedd et al., 1999). A longitudinal study found that the genu of the CC was larger in males compared to females with age-related changes found in the splenium between the ages of 5 and 18. Hemispheric differences were also found. The right hemisphere and right caudate volumes were larger than the left for both genders (Giedd et al., 1999). In contrast, the left lateral ventricles and putamen were larger than the right for both genders, showing consistent asymmetries for males and females.
Many of the neuroimaging differences seen may differentiate children with various psychiatric disorders from typically developing children. The regions involved in attention, inhibition, reading, and language are all areas that have been found to differ in males with ADHD and/or reading disabilities. The following section briefly discusses the work that has been completed with males with these disorders. The interested reader is also referred to the chapters within this volume on learning disabilities and ADHD for further information.
Gender Differences in Disorders Diagnosed in Childhood
Differences in genetics, hormones, and environmental effects contribute to developmental differences in brain function and size that may differentiate children with various psychiatric and learning disorders. Specifically, the regions involved in attention, inhibition, reading, and language are specific areas found to differ in children and adolescents with ADHD and/or learning disabilities. Additionally, recent studies indicate there may be specific differences in the brains of males and females diagnosed with autism spectrum disorders (ASDs) (Doyle-Thomas et al., 2014; Groen et al., 2008).
Neuroimaging in Males with ADHD
Many gender-specific research findings are of interest in children with ADHD. However, this disorder has been found to have a higher incidence for males, and the majority of theories and published research has been based on samples consisting primarily of boys. Psychosocial impairment in girls with ADHD is also well established. On the other hand, the neuropsychological and neurobiological basis of these deficits is less frequently examined (Mahone & Wodka, 2008). Many studies that include small samples of girls preclude the ability to examine the findings in a sex-specific manner. Some preliminary data is available. In a longitudinal study Shaw et al. (2009) found that children with ADHD show relative cortical thinning in regions important for attentional control, with those with a predicted worse outcome having a fixed thinning of the left medial prefrontal cortex, which may compromise the anterior attentional network and hamper clinical improvement. Right parietal cortex thickness normalization in patients with a better predicted outcome was hypothesized to represent compensatory cortical change, although growth curves for both males and females were similar in developmental trajectories of regional brain volumes. For most regions of interest examined (including total cranial volume and cerebellum, but not caudate), the growth curves of children with ADHD (relative to controls) were parallel, but on a lower track. Additionally, there was no interaction between diagnosis and sex, implying that both males and females with ADHD show stable reductions in brain volume as compared to controls (Castellanos & Tannock, 2002). While this study included children as young as 4 years, most participants were of school age. Therefore, it is difficult to draw conclusions about the early trajectory of anomalies that may be associated with ADHD.
Child and adolescent males with ADHD have been found to show smaller caudate volumes (Filipek et al., 1997; Semrud-Clikeman & Pliszka, 2006). Studies in ADHD have found smaller volumes specific to the right caudate, in addition to smaller volumes of the right frontal lobe, right globus pallidus, and left cerebellum in males but not females (Castellanos et al., 1996). These consistent gender-based differences have been found in the cerebellum and in total brain volume for both genders with ADHD. Additionally, it has been suggested that females and males use different behaviorally relevant neurocognitive strategies. Several studies have found greater right prefrontal cortex activation in females. However, the spatiotemporal dynamics of neural events associated with these sex differences remains unclear. One event-related potential (ERP) study examined the neural substrates of human cognitive sex differences elicited by a visual attention challenge (an area of deficit often associated with ADHD) (Neuhaus et al., 2009). Equal numbers of male and female participants (22) matched for age, education, and nicotine use were studied with 29-channel-electroencephalography recorded under a visual selective attention paradigm, the Attention Network Test. Visual ERPs were topographically analyzed and neuroelectric sources were estimated. This analysis revealed a novel frontal-occipital second peak of the visual N100 that was significantly increased in females relative to males, as well as a corresponding central ERP component, that was recorded in females exclusively, at around 220 ms. Thus, this study found a strong sex-based difference in the relationship between stimulus salience and central ERP component amplitudes. Subsequent source analysis revealed increased cortical current densities in right rostral prefrontal (BA 10) and occipital cortex (BA 19) in female subjects that was not found in males. This study was the first to report a three-part association between sex differences in ERPs, visual stimulus salience, and right prefrontal cortex activation during attentional processing and suggests that anatomical differences exist between males and females with ADHD.
Cerebral glucose metabolism studies (using positron emission tomography (PET) scans) have also been used to investigate differences in males and females with ADHD. However, studies have taken place with small sample sizes, so caution should be exercised when interpreting the results. These studies have indicated that girls with ADHD may show decreased cerebral glucose metabolism compared to girls without ADHD, with decreased metabolism in the left anterior frontal lobe significantly correlated with ADHD symptom severity (p < 0.001). While sample sizes have been small, the findings and hypotheses have been replicated, indicating adolescent girls with ADHD show less activation compared to healthy control girls, and less global cerebral glucose metabolism than boys with ADHD (Mahone & Wodka, 2008).
One complication in understanding ADHD is that it is considered a developmental disorder that, by current definition, has onset prior to 7 years of age. Imaging studies have provided some insight into brain differences, but thus far have almost exclusively focused on children 7 years of age and older. Recently, Mahone et al. (2011) completed a study using high-resolution anatomical (MPRAGE) images to better understand the neurobiological development of ADHD in younger children presenting with symptoms of the disorder, while controlling for language disorders. Cortical regions were delineated and measured in the study using automated methods in Freesurfer, while basal ganglia structures were manually delineated. Results indicate that even younger children with ADHD show significantly reduced caudate volumes bilaterally. However, there were no significant group differences in cortical volume or thickness in this age range. Furthermore, results indicated that, after controlling for age and total cerebral volume, left caudate volume was a significant predictor of hyperactive/impulsive behavior, but not inattentive symptom severity. Although results are not gender-specific, they suggest that irregularities in basal ganglia, particularly caudate development, appear to play an important role in children presenting with early onset symptoms of ADHD (Mahone et al., 2011).
There is a substantial literature demonstrating that the neuropsychological and neurobiological basis of ADHD in children is associated with globally decreased total brain and cerebellar volumes and striatal volumetric abnormalities that are age-dependent. However, these studies typically do not contain a large enough sample to determine if findings are sex-dependent. A previous prospective study of lobar volumes in children with ADHD was reexamined using a measure of cortical thickness (Shaw et al., 2009). Findings indicated children with ADHD had global thinning of the cortex that was most prominent in the medial and superior prefrontal and precentral regions important for attentional control. Although not sex specific, the children with worse clinical outcomes had a fixed thinning of the left medial prefrontal cortex, which may compromise the anterior attentional network and deter clinical improvement. Cortical thickness developmental trajectories did not differ significantly between the ADHD and control groups except in the right parietal cortex, where trajectories converged, which occurred only in the better outcome group. This right parietal cortex thickness normalization may represent compensatory cortical change. However, further study is needed to determine whether these effects are sex specific.
The mechanisms underlying the increasingly recognized motivation deficits that contribute to ADHD are being examined through neuroimaging. Tomasi and Volkow (2011) compared the functional connectivity density between 247 ADHD and 304 typically developing control children from a public MRI database. Results of their analysis indicated enhanced connectivity within reward-motivation regions and decreased connectivity with regions from the default-mode and dorsal attention networks. Again, while not sex specific, this study suggests impaired interactions between control and reward pathways in ADHD that may contribute to both attention and motivation deficits.
Increasingly sophisticated methods examining cortical topography, cortical thickness, or voxel-based morphometry, as well as neuroimaging techniques that provide a means to uncover the neurobiological mechanisms by which gene variants impact the brain, are beginning to better demonstrate more clearly delimited anatomic abnormalities. The overall pattern of results from the more sophisticated imaging techniques is not clearly convergent, potentially suggesting heterogeneity in ADHD (Castellanos et al., 2009). Models focusing on intra-subject variability, intrinsic brain activity, and reward-related processing are progressing rapidly and should provide clearer understanding of the multiple types of ADHD, as well as a better understanding of variations of connectivity contributing to differences between ADHD processing anomalies in males and females. Additional studies focusing on how genetic changes can affect brain structure, chemistry, and function are also promising (Durston, 2010).
In conclusion, findings suggest that lower glucose metabolism is more characteristic of girls with ADHD. However this has not been tested with samples that control for sexual maturation, cognitive ability, and genetic relatives with ADHD. Additionally, studies have shown that both males and females with ADHD show stable reductions in brain volume as compared to controls, with males with ADHD found to show smaller caudate volumes. Emerging imaging techniques, particularly those based on the approach of resting-state functional connectivity, and genetic neuroimaging, appear to be promising in better understanding gender differences for the disorder, as well as offering a possible means of addressing the complex heterogeneity of ADHD as testable models of pathophysiology are developed.
Neuroimaging in Males with Learning Disabilities
While there is now an informative body of research on the use of neuroimaging techniques in revealing brain morphometric differences in people with learning disabilities (Altarelli et al., in press; Hynd & Semrud-Clikeman, 1989), most differences have generally been studied with either a mixed gender sample or with males only. These studies have typically been focused on the language regions of the brain, and language laterality and degree of difference has varied among the studies due to methodological issues. Most studies have not controlled for sex and have thus provided little information about possible sex-specific variations in brain structure and function in children with learning disabilities. However, with less invasive techniques becoming available, younger children are now beginning to be included in study samples.
Language acquisition, strongly tied to learning ability, is inherent in changes that occur in the rapidly developing young brain. During the years of language acquisition, the brain not only stores linguistic information but also adapts to the grammatical regularities of language. Advances in functional neuroimaging have substantially contributed to systems-level analyses of brain development, including overall language acquisition, including reading language, and understanding of the contributions of cortical plasticity for second language acquisition (Sakai, 2005). There is about a 2.5 to 3:1 gender difference in dyslexia (a very broad term defining a learning disability that impairs a person’s fluency or comprehension accuracy for reading) for boys to girls, with studies showing females have more cell density in the planum temporale than males (Witelson, Glezer, & Kigar, 1995; Witelson & Kigar, 1988a, 1988b, 2004; Witelson & McCulloch, 1991). Given the lower incidence of dyslexia in girls, it may be that this cell density is protective for reading and written language problems in females.
More recent neuroimaging studies investigating the neural correlates of verbal fluency (VF) focused on sex differences. It has been hypothesized that reading disabled children have a domain-general deficit in processing rapidly occurring auditory stimuli that degrades speech perception, thereby limiting phonologic awareness and so, reading acquisition. This was not proved to be the case in a study with 100 7–11-year-old children with learning disability and 243 non-learning disabled children who were evaluated on a two-tone auditory discrimination paradigm (Waber et al., 2001). Those with a learning disability committed more errors, but effects of timing were comparable to their non-disabled peers. The same result was obtained for a subsample of good and poor readers. The authors concluded that task performance did predict reading, spelling, and calculation ability. However, while neural processes underlying perception of speech and other auditory stimuli may be less effective in poor readers, rate appears to not be specifically affected regardless of sex.
Another study focusing on fluency rate found activation in the inferior frontal gyrus (IFG), insula, anterior cingulate cortex (ACC), medial frontal gyrus (mFG), superior (SPL) and inferior parietal lobules (IPL), inferior visual areas, cerebellum, thalamus, and basal ganglia (Gauthier et. al., 2009). This study was completed with right-handed, French-speaking, male (172) and female (159), college-age subjects performing a phonological verbal fluency task. Results indicated males, more than females, activate several regions involved in mental imagery strategies linked to word generation, including the lingual gyrus, inferior temporal gyrus (ITG) and posterior cingulate, in addition to one region linked to performance monitoring (the ACC), and other areas associated with the implementation of top-down control processes (right superior frontal gyrus (SFG) and right, dorsolateral prefrontal cortex (DLPFC)), as well as the cerebellum. Thus, findings indicated activity in three discrete subregions of the ACC related to sex, performance and their interaction, respectively. In addition to these differences, results show males seem to require greater use of visual mental strategies, subserved specifically by the precuneus, to reach a verbal fluency level equivalent with that of high-scoring females. High-scoring men also were found to solicit more areas in the left hemisphere implementing top-down control processes (DLPFC). Regardless of sex, a low level in verbal fluency scores related to greater activity in the dorsocaudal ACC. In females only, the low performance level is associated with a stronger activation in a more rostral part of the dorsocaudal ACC, which reinforces the role of the ACC in general performance monitoring. Findings also indicated that the cerebellum seems to contribute to the high verbal fluency performance level independently from sex.
Another investigation examined the impact of subject age, language task, and cortical region on the occurrence of sex differences in functional MRI with 205 (104 male, 101 female) right-handed, monolingual, English-speaking children between the ages of 5 and 18 (Plante et al., 2006). The study used fMRI at 3T to evaluate BOLD signal variation associated with sex, age, and their interaction. Brain activation in classical, left hemisphere language areas of the brain and their right homologues were assessed for sex differences. For this study, children completed up to four language tasks, which involved listening to stories, prosody processing, single word vocabulary identification, and verb generation. Sex difference for behavioral performance was found for the prosodic processing task only. Left lateralization was present for both frontal and temporal regions for all but the prosody task, although no significant sex differences were found for the degree of lateralization.
Although sex differences in visuospatial processing, also thought to contribute to learning difficulties, are a consistently robust finding in adults, few neuroimaging studies have examined this issue in younger populations. Clements-Stephens, Rimrodt, and Cutting (2009) used functional neuroimaging (MRI) to examine whether sex-based differences in visuospatial processing exist in children, and/or if they develop during childhood. Thirty-two participants, between 7 and 15 years of age (16 males, 16 females) matched on performance, participated in this study. Overall, both groups showed overlapping activation in the superior parietal lobes bilaterally, extrastriate cortex, and cerebellum. Males had significantly greater activation in the cerebellum and right lingual gyrus. Formal comparisons between age groups indicated older males show engagement of left hemisphere regions, while females show greater engagement of right hemisphere regions traditionally associated with visuospatial processing. Results also suggest older males, as compared to younger males, may engage regions that are associated with a visuomotor network, whereas females may tend to use areas indicated in spatial attention and working memory to complete visuospatial tasks. Furthermore, results suggest that differential engagement of networks associated with visuospatial processing may be due to differences in strategy use that are evident early on and continue to develop over time.
Connectivity neuroimaging studies are also beginning to be conducted with younger children, and appear to support the view that a left-lateralized brain network is crucial for language development in children. Kikuchi et al. (2011) used a custom-sized magnetoencephalography (MEG) system, a noninvasive brain imaging technique that is a practical neuroimaging method for use in young children, to investigate brain networks of 78 right-handed preschool children (32–64 months) while they listened to stories with moving images. MEG produces a reference-free signal, and is therefore a useful tool to compute coherence between two distant cortical rhythms. Results indicated that left dominance of parietotemporal coherence in theta band activity (6–8 Hz) was specifically correlated with higher performance of language related tasks. This laterality was not correlated with nonverbal cognitive performance, chronological age, or head circumference. These results suggest that it is not the left dominance in theta oscillation per se, but the left-dominant phase-locked connectivity via theta oscillation that contributes to the development of language ability in young children.
The growing body of evidence that male and female brains develop and mature at different rates (Holland et al., in press) suggests that early differences in brain development likely contribute to learning differences that may also be sex-specific. Much larger numbers of subjects would be needed to confirm findings from neuroimaging studies involving learning disabilities. However, it does appear that over time, males develop a more integrated visuomotor/visuospatial network while females appear to develop a more spatial attention/working memory system for learning. The differences in activation patterns in females may be mediated by a strong verbal strategy used early in development. Additionally, differences seen in males are consistent with visually based strategy use in which it appears that males may rely on imagery and are more hands-on when completing tasks. Males’ reliance on a visuomotor network may also account for the traditional advantage reported on visuospatial tasks. The differential engagement of different networks associated with visuospatial processing between males and females seen with more complex tasks (such as mental rotation) seems to be supported, which could reflect a true sex-based difference in visuospatial processing that may affect learning in general. Also, emerging research suggests that the in utero experience of testosterone may affect functional asymmetries and could be linked to some differences in learning. These areas of research, as well as the relationship of handedness and/or age effects in differences of area and volume for key structures, are variables that require additional study across sexes. Additionally, there are very few studies that include sufficient numbers of minorities, and the possible effects of ethnicity on brain development have not been explored sufficiently. As these students are often over-identified for special education remediation, there may be even more reason to develop gender-specific research in this area.
Neuroimaging in Males with Autism
Characteristics that are attributed to autistic children, including impairments in social interaction, deviations in language usage, and restricted and stereotyped patterns of behavior have been found regardless of age, IQ, and gender. However, brain changes due to age, IQ, and gender may pose potential confounds in autism neuroimaging analyses (Kurth et al., 2011). Causes and contributing factors for autism continue to be poorly understood. The prevalence of children identified with autism is rising with the most recent data from the Center for Disease Control (2006) indicating an average of 1 in 110 children in the United States being diagnosed with autism. The most current information suggests that genetic and environmental factors contribute etiologically to this disorder. Data gained from twin, family, and genetic studies support a role for an inherited predisposition; with, clinical, neuroanatomic, neurophysiologic, and epidemiologic studies suggesting that gene penetrance and expression is likely influenced by the prenatal and early postnatal environment (Hertz-Picciotto et al., 2006). Some studies link autism to xenobiotic chemicals and/or viruses, including the CHARGE (Childhood Autism Risks from Genetics and Environment) project, begun in 2003 to investigate underlying environmental and genetic causes for autism and triggers of regression and to systematically study a wide spectrum of chemical and biologic exposures, susceptibility factors, and their interactions (Hertz-Picciotto et al., 2006). To date, CHARGE is the largest and most comprehensive assessment of children with autism ever undertaken. It aims to distinguish subgroups, or phenotypes, of autism based on thorough biomedical and behavioral analyses of affected children. A wide variety of studies have resulted from the ongoing CHARGE project, including the launch of another comprehensive autism-focused venture, the Autism Phenome Project (APP). However, little of the derived information is sex-specific.
Others have begun to research the relatives of people with autism who show milder expression of traits for the disorder, referred to as the Broader Autism Phenotype (BAP). Several neurofunctional and neuroanatomical studies of autistic individuals and their relatives using neuroimaging techniques such as fMRI, MRI, EEG, MEG, and DTI were compiled to examine important differences in brain structure, activity and connectivity in and between regions of the brain (Sucksmith, Roth, & Hoekstra, 2011). This extensive examination of the neural substrates of the BAP contributes to our overall understanding of the disorder, and helps to delineate better the possible heritable endophenotypes so that autism susceptibility can be more reliably indexed. The compiled research, although again not sex-specific, also adds to our understanding of the neural correlates of the cognitive aspects of autism (e.g., sensory perception, social cognition, and visual attention), and contributes toward a solution that will bridge the gap between genes and clinical autism diagnosis.
One hallmark symptom for children with autism includes deficits in the perception of social stimuli that may contribute to the characteristic impairments in social interaction. The cortical processing of voice is abnormal in even high-functioning autistic children (Groen et al., 2008). Significant differences in response time to the perception of a voice are noted with children with autism responding much more slowly compared to control children. No gender differences were found for either the autistic or control samples.
Another area of perception of social stimuli that may contribute to the characteristic impairments in social interaction for autistic children is the ability to understand and respond to emotions. Most neuroimaging studies that have reported gender differences in response to human emotions have used face photographs. One exception is a study completed by Fine, Semrud-Clikeman, and Zhu (2009) that used human face photographs of positive and negative emotions, along with video vignettes of positive and negative social human interactions, in an attempt to provide a more ecologically appropriate stimuli paradigm. Although this study involved healthy, non-autistic male and female young adults (ten each), findings contribute to the overall understanding of sex-specific differences in processing emotion. Using conservative ROI (region of interest) analysis, the authors found greater male than female activation to positive affective photographs in the anterior cingulate, mFG, SFG and superior temporal gyrus, all in the right hemisphere, with no significant ROI gender differences observed for the negative affective photos. More activation was noted in ROIs of the left posterior cingulate and the right ITG to positive social videos, and in the left middle temporal ROI for negative social videos for males as compared to females. Additionally, males were more lateralized than females. Furthermore, although more activation was observed overall to video compared to photograph conditions, males and females appear to process social video stimuli more similarly to one another than they do for still photos. As males are four times more likely to be diagnosed with autism, it may be that further research can expand on this information concerning differences in processing human emotion that may be useful in understanding sex-specific differences in emotional processing between autistic males and females.
Connective imaging has also been employed with autistic subjects. As autism has been hypothesized to reflect neuronal disconnection, several recent studies have examined key thalamic relay nuclei and cortico-thalamic connectivity in the pathophysiology of the disorder. One study, using DTI with 17 boys with ASD and 17 typically developing controls, produced results (using whole-brain voxel-wise analyses) that evidenced disturbances in the thalamo-frontal connections. These findings, although found in a sample that includes only males, so cannot be noted as sex-specific, emphasize the role of hypoconnectivity between the frontal cortex and thalamus in autistic children (Hong et al., 2011).
Imaging studies have also examined genetic variation in ASDs, particularly the oxytocinergic system that may modulate sociality and indicate risk for social dysfunction (Tost et al., 2010). Oxytocin, centrally released, facilitates offspring survival by initiating mother–infant bonding and the onset of maternal behavior. The neural architecture of the oxytocinergic system targets a variety of brain areas, but for children with autism, the most salient are those critical for emotion regulation (e.g., amygdala, lateral septum, and brainstem) (Lee et al., 2009). Given the known heritability of social behavior in humans, it is possible that the genetic variation in the oxytocinergic system may modulate sociality and contribute to risk for social dysfunction. Consequently, common variants in the oxytocin receptor gene (OXTR) have been examined in the context of risk for ASD. Toth et al. (2007) used a multimodal imaging intermediate phenotype approach to show that a common genetic variant in OXTR linked to social function predicts individual differences in brain structure, brain function, and personality in healthy humans. The same study provided evidence for a sex-dependent impact of OXTR genotype on limbic structures related to prosocial temperament. Together, these findings indicate a neural mechanism for genetically increased risk of social impairment, predominantly in males, that seems to be of potential relevance for autistic (and possibly other psychiatric) disorders. Other connectivity imaging studies using DTI and diffusion tensor tractography have been conducted with findings implicating the corpus callosum (Hong et al., 2011) and the cerebellar peduncles (Brito et al., 2009). Additionally, morphometric measures of the basal ganglia and thalami in 3–4-year-old children have been studied which indicate that rigid social behavior (repetitive behavior), common early in the clinical course of ASD, may be associated with decreased volumes of the basal ganglia and thalamus. Although these studies indicate reduced connectivity in corpus callosum, internal capsule, and superior and middle cerebellar peduncles and reduced volumes in the basal ganglia and thalamus as compared to the findings in age-matched healthy children, they still do not control for sex, and so it is unknown whether these findings manifest differently in males and females with autism.
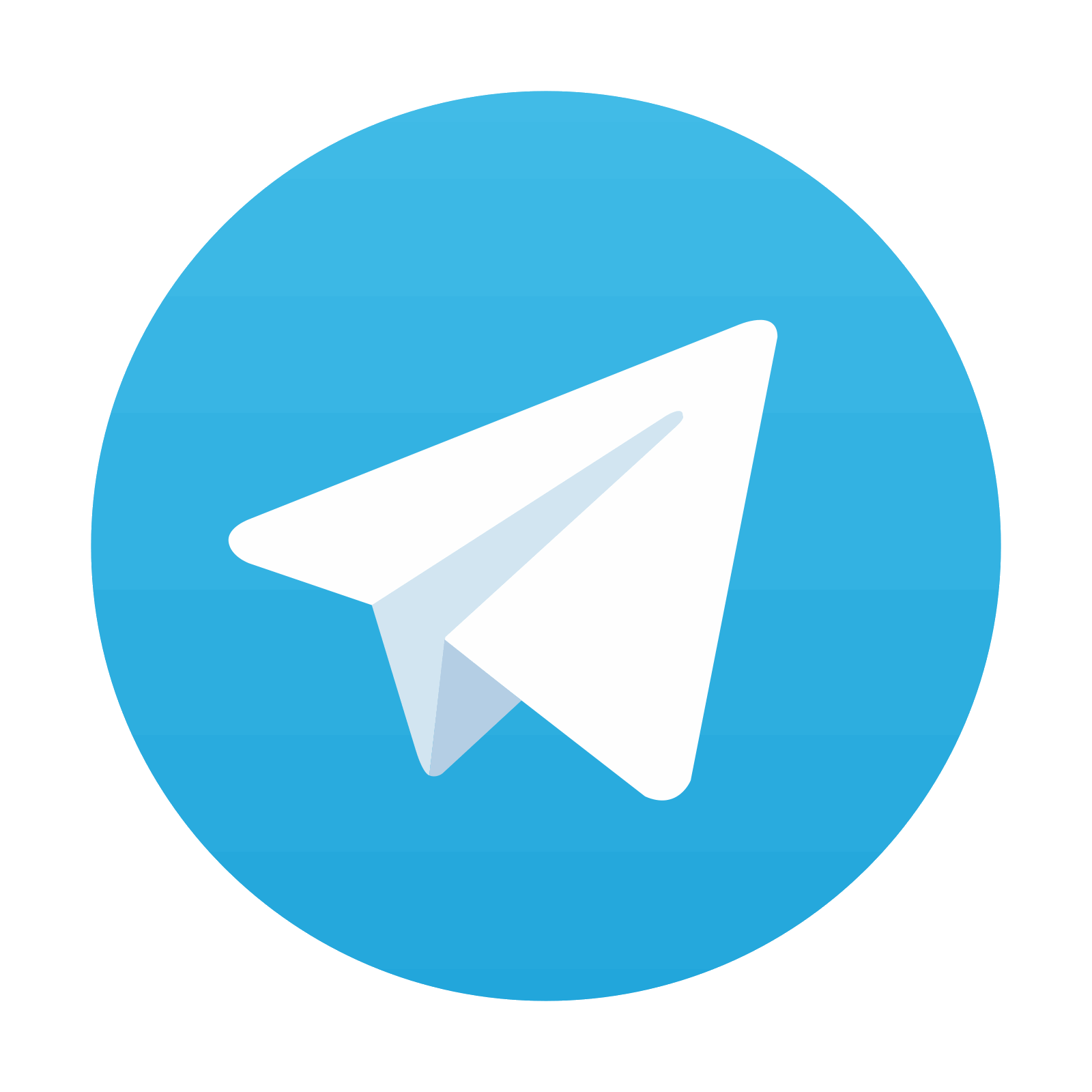
Stay updated, free articles. Join our Telegram channel
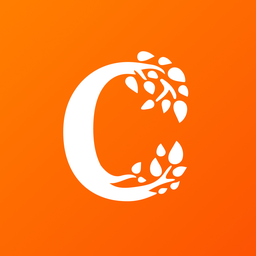
Full access? Get Clinical Tree
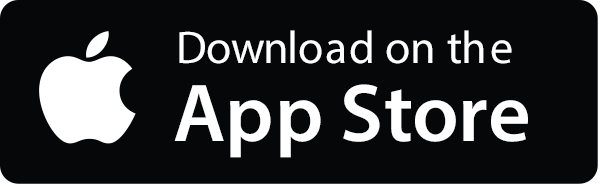
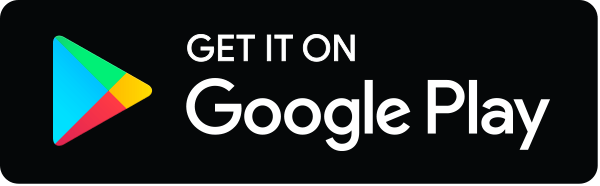