Fig. 1
Functional neuroanatomy of human REM sleep. Brain regions more activated during REM sleep are indicated with an upward red arrow, and those less activated are indicated with a downward blue arrow. Data from studies using PET imaging (Braun et al. 1997, 1998; Maquet et al. 1996, 2000; Nofzinger et al. 1997). PPT penduculopontine tegmental nuclei. Adapted from Perogamvros and Schwartz (2012)
Taken together these studies suggest substantial activation of emotional networks during all stages of sleep that may be more persistent and less variable during REM sleep than during NREM sleep.
2.2 Activation of Reward Networks
The reward system contributes to the development and monitoring of goal-directed and adaptive behaviors in all mammals, including humans. The reward system is responsible for the processing of rewards or punishers, which typically serve respectively as positive or negative signals for behavioral adaptation and learning. In this way, reward processing is often associated with the feeling of anticipation while seeking a reward and the pleasure when obtaining it. On the other hand, punishers can induce emotional states such as anger or fear and elicit withdrawal behavior. In addition to brain regions involved in emotional regulation, brain areas that are more specifically involved in the regulation of reward are also activated during sleep , including the ventral tegmental area (VTA) and the nucleus accumbens (NAcc) (see Fig. 3). These activations during sleep likely contribute to the reprocessing of memories with a high emotional or motivational relevance, in coordination with emotion-related circuits (for a review, see Perogamvros and Schwartz 2012).
Two studies have demonstrated that dopaminergic neurons in the VTA of rats increase their bursting activity during REM sleep, which results in a large synaptic dopamine release in the NAcc shell (Dahan et al. 2007; Maloney et al. 2002). This activity is significantly higher during REM sleep as compared to NREM sleep and relaxed or quiet wakefulness. In fact, VTA activation during REM sleep is comparable in intensity and duration to VTA activation during feeding or sex. A second important region for reward processing is the NAcc, whose activity is typically greatest for large rewards (Cooper and Knutson 2008). Activity in the NAcc is increased during REM sleep (Lena et al. 2005). Other reward-related regions, like the ventromedial PFC (Haber and Knutson 2010) and the ACC, which assign a positive or negative value to future outcomes (Bush et al. 2002; Takenouchi et al. 1999), are activated during REM sleep, in both animals and humans (Lena et al. 2005; Maquet et al. 1996, 2000). In addition, neuronal activity in the hippocampus displays a theta rhythm (i.e., an oscillatory pattern in EEG with a frequency range of 5-12 Hz in behaving rats and 4-7 Hz in humans) during REM sleep in both animals (Popa et al. 2010; Winson 1972) and humans (Cantero et al. 2003) studies. This latter finding is remarkable considering that increased theta oscillations have been reported during novelty-seeking, exploratory and instinctual behaviors, which are also seen in REM sleep behavior disorder and other parasomnias (Perogamvros et al. 2012). Finally, the orexin/hypocretin neurons in the lateral hypothalamus have transient discharges during REM sleep (Mileykovskiy et al. 2005; Takahashi et al. 2008). The orexin/hypocretin system is particularly interesting because it plays a key role in both the regulation of sleep –wake states and emotional/motivated behaviors, via strong functional interactions with the mesolimbic dopamine circuitry (Harris et al. 2005; Ponz et al. 2010a, b; Schwartz et al. 2008; Thompson and Borgland 2011).
Activation of reward-related regions is, in some cases, also observed during NREM sleep. In particular, a spontaneous reactivation (or replay) of neuronal firing patterns occurs in the ventral striatum of rats after a reward searching behavior (Lansink et al. 2008; Pennartz et al. 2004). This off-line replay is induced by hippocampal ripples and seems to be related to linking a memory trace to a motivational or emotional value during sleep (Lansink et al. 2009). Whether a similar neural mechanism exists in humans is still unclear, but brain imaging studies have demonstrated that the hippocampus and the ventral striatum are also activated during NREM sleep (Nofzinger et al. 2002), and that increased hippocampal activity may relate to subsequent performance improvement (Peigneux et al. 2004). Some other reward-related regions are also found to be activated during NREM sleep, like the posterior insula, ACC (Schabus et al. 2007), and the amygdala (Nofzinger et al. 2002).
3 Implications of Emotional/Motivational Networks Activation during Sleep
3.1 Emotional Maturation
The function of the brain which is most influenced by sleep is the memory; not that it entirely ceases; but it is brought back to a condition of imperfection, such as everyone may have experienced in pre-historic times, whether asleep or awake (Friedrich Nietzsche, 1878, “Human, All Too Human”, section one: “Of First and Last Things”, aphorism #12).
According to Nietzsche, the mind in sleep regresses to an archaic state of waking reasoning and consciousness, resembling that of early mankind. More than one century later, Jaak Panksepp claimed that an archaic affective state is expressed in REM sleep and dreams (Panksepp 1998), while Allan Hobson suggested that REM sleep is a protoconsciousness state preceding the maturity of the waking consciousness (Hobson 2009, see also Koukkou and Lehmann 1993).
Recent studies support the role of sleep in emotional maturity of the human. Since as early as the 13th week of gestation and neonatal period, smiles and other expressions are extremely common, especially during active REM sleep (Dondi et al. 2007; Emde and Koenig 1969; Messinger et al. 2002). The recent work of Messinger and collaborators showed that neonatal smiles, in particular Duchenne smiles that involve cheek raising and index positive emotion (Ekman 1992), are more frequent, stable, and enduring during active sleep (corresponding to adult REM sleep, Roffwarg et al. 1966) than during other behavioral states (Dondi et al. 2007; Messinger and Fogel 2007) (Fig. 2). These authors put forward the interesting hypothesis that such smiles could relate to the activation of the amygdala and limbic structures during REM sleep (see Sect. 2 above), and may foster the functional coordination of facial motor programs with neural structures supporting the emotional and social expression of smiling later in development (at about 2 months of age) (Dondi et al. 2007).
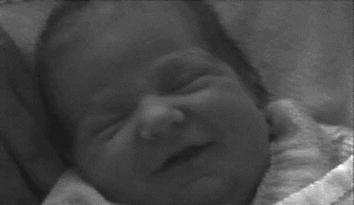
Fig. 2
Smile of a 42-hour-old sleeping infant. Typical Duchenne smile with cheek raising which is thought to relate to the experience of positive emotion (see main text). Reproduced from Messinger et al. (2002) with permission
Recent imaging data in 3- to 7-month olds infants also revealed specific brain responses to emotional human vocalizations during sleep, involving the orbitofrontal cortex and insula (Blasi et al. 2011). Furthermore, it is noteworthy that active (or REM) sleep occupies the biggest portion of a child’s sleep and occurs much earlier in human development than proper dreaming (Foulkes 1993). Altogether, these data suggest that REM sleep of infants is likely to subserve crucial emotional (Berger et al. 2012; Perogamvros 2012) and learning functions (Fifer et al. 2010), much like what is found in adults (Sterpenich et al. 2009; Wagner et al. 2001) (see Sect. 3.5). However, these data should not eclipse the possible contribution of sleep structure and NREM sleep (quiet sleep in newborns) to these important functions. Indeed, a recent study tested whether sleep–wake transitions in the neonatal period might predict emotional and cognitive development in premature infants during the first 5 years of life (Weisman et al. 2011). Sleep states at a gestational age of 37 weeks, emotional regulation during infant–mother and infant–father interactions at 3 and 6 months, cognitive development at 6, 12, and 24 months, as well as verbal IQ, executive functions, and symbolic competence at 5 years were assessed. It was shown that sleep-state transitions characterized by shifts between quiet sleep and wakefulness (contrary to rapid cycles between states of high arousal, such as active sleep and cry) are important for an optimal emotional and cognitive development, at least in premature infants.
3.2 Dreaming
Recent empirical research on dreaming favors a relatively broad definition of dreaming as corresponding to any internally generated sensory, motor, emotional or cognitive subjective experiences that occur during sleep. Our neurobiological knowledge about human dreaming derives primarily from the study of REM sleep, because this sleep stage has initially been linked to dreaming activity (Dement and Kleitman 1957). However, dreaming may occur in any sleep stage (i.e., both REM and NREM sleep stages) (Foulkes 1996; Oudiette et al. 2012), although distinct phenomenological characteristics differentiate REM from NREM dreaming. For example, dream reports are on average more vivid, bizarre, with complex narratives, i.e., more ‘dream-like’, after awakenings from REM sleep than from NREM sleep (Fosse et al. 2001; Strauch and Meier 1996). In addition, REM dreaming is characterized by content with emotional and motivational value (e.g., socializing, fighting, or sexual activity). Note that daily routine activities such as typing, washing dishes, or buying food at the supermarket are generally not frequent in dream reports (Schredl 2010). Motivational and emotional content is more prominent in REM than in NREM dreaming (Smith et al. 2004). This is consistent with the finding that several limbic and mesolimbic dopaminergic regions are selectively activated during REM sleep, amygdala activity and burst firing in the VTA being significantly higher in REM sleep compared to NREM sleep. Moreover, lesion studies abolishing REM sleep muscle atonia in cats, revealed a predominance of seeking (e.g., spatial exploration) and emotional behaviors (e.g., anger) during enacted behaviors in REM (or paradoxical) sleep (Jouvet 1965). Similarly, aggressive behaviors, but also seeking types of behaviors (such as mimicking eating, drinking, smoking a cigarette, etc.), are also frequent in patients with REM sleep behavior disorder, who act out their dreams (Oudiette et al. 2009).
To account for the high prevalence of fear-related experiences in dreams, the psychologist and philosopher Revonsuo (2000) proposed the Threat Simulation Theory, according to which dreaming allows an organized and selective offline simulation of threatening events that promotes the development and maintenance of threat-avoidance skills during wakefulness. Realistic threatening experiences in a dream would thus activate threat-related brain circuits, in particular the amygdala, and would lead to improved performance in real life situations (Valli and Revonsuo 2009). Interestingly, individuals suffering from post-traumatic stress disorder simulate threatening events in their dreams more often than controls (Valli et al. 2005), and REM sleep deprivation impairs threat avoidance skills in waking life (Martinez-Gonzalez et al. 2004). Actually, it has been proposed that experiencing threatening stimuli (objects, situations, thoughts, memories, and physical sensations) in a totally safe context during dreaming may resemble exposure therapy for anxiety disorders (Desseilles et al. 2011; Pace-Schott et al. 2009). Nightmares such as in post-traumatic stress disorder would by contrast reflect the failure of an adaptive fear memory extinction process, in the presence of temporary (e.g., daily concerns) or more persistent (e.g., trauma) increases in affect load (Nielsen and Levin 2007). Yet, whether the off-line reprocessing of emotional information requires conscious experience such as in dreaming remains unresolved.
We previously proposed the Reward Activation Model for sleep and dreaming (Perogamvros and Schwartz 2012), according to which emotional memories are prioritized for offline reprocessing (see Sect. 3.5) through the activation during sleep (and not necessarily in dreams) of the SEEKING system. The latter corresponds to an emotional and motivational system of the mammalian brain, which is related to approach behaviors and to the intense feeling of anticipation while seeking a reward (Panksepp 1998). More specifically, sleep would favor the activation of stimulus representations or behaviors of high emotional and motivational relevance, which induce instinctual behaviors or drives (such as feeding, mating, fighting, fleeing, etc.), as well as approach and avoidance behaviors. In our model, emotionally relevant experiences (including threat-related information) have a higher probability of being activated during sleep and have a preferential access to sleep-related memory consolidation processes. It is thus proposed here that one of the main functions of dreaming is to expose the sleeper to rewarding or aversive stimuli, in order to maintain and improve offline memory consolidation processes and performance in real life situations, while also contributing to emotion regulation processes. The reader may refer to Perogamvros and Schwartz (2012) for a detailed description of the Reward Activation Model.
3.3 Sexuality in Sleep
Surprisingly, although sexual content is prominent in dreams (Freud 1900; Schredl 2010), to our knowledge no neurophysiological interpretation of sexual dreaming has yet been proposed. The sexual content of dreams can be regarded as an expression of the activation of reward -related functions during sleep and thus be viewed under the scope of the Reward Activation Model (Perogamvros and Schwartz 2012). Mesolimbic activation during sleep would indeed create a favorable neurophysiological environment for the elicitation of approach behaviors, including those triggered by primary rewards such as sex. Recent neuroimaging studies on sexual stimulation and orgasm in humans confirmed activations across reward and emotional networks (VTA, striatum , insula), but also revealed simultaneous deactivations in structures related to monitoring and cognitive control (prefrontal and orbitofrontal cortex) (Georgiadis et al. 2007; Holstege and Georgiadis 2004; Holstege and Huynh 2011). Notably, these activation/deactivation patterns are also typically observed during REM sleep (see Sect. 2 above). Therefore, activation of reward-related structures (VTA , insula) may favor the elicitation of primary rewards, including sex, in the dreams, whereas deactivation of attentional and cognitive control structures (e.g., parietal cortex, dorsolateral PFC; Sect. 2.1) and the partial disconnection from external sensory inputs, may support relaxation of sexual behavior from social or moral constraints.
Interestingly, testosterone levels in humans increase during the first part of the night, peaking during the first REM periods, and are then stable until awakening (Luboshitzky et al. 1999). This hormonal modulation depends on sleep and not on circadian factors since testosterone levels in young men rise during daytime sleep just as in nighttime sleep, while the levels fall upon waking (Axelsson et al. 2005). The picture is much less clear for women because of the complexity of measuring hormonal levels across the menstrual cycle and many other confounding factors, such as the use of birth control pills. The data available so far point to a direct effect of sleep on the activity of the neuroendocrine reproductive control, ultimately improving sexual satisfaction (Andersen et al. 2011).
Taken together these findings suggest that increased dopaminergic and hormonal activity, and decreased PFC monitoring may contribute to both promoting sexually relevant content in dreams and eliciting sexual arousal during REM sleep. Yet, future correlation studies among dream content, sexual arousal, and hormonal activation are needed to clarify whether the occurrence of spontaneous sexual arousal during REM sleep (Bancroft 2005; Fisher et al. 1983; Karacan et al. 1975) and sexual dreams are independent expressions of a common underlying neurophysiological mechanism (Lamid 1986), whether sexual content of dreams may trigger physiological sexual arousal, or whether the latter may infiltrate the content of the dreams.
3.4 Insight and Creativity
The role of sleep in problem solving and creativity is more than a folk belief. Indeed, in addition to its deleterious impact on reward and emotional functions (Sect. 4), sleep deprivation (SD) impairs affectively guided decision making (Pace-Schott et al. 2012), moral reasoning (Killgore et al. 2007), insight (Wagner et al. 2004), and creativity (Cai et al. 2009; Home 1988). Based on the ‘carry-over effect’ after awakening (during which performance is altered as a result of the brain’s slow transition to full wakefulness), elegant studies by Stickgold and colleagues showed that subjects awoken from REM sleep had a 32 % advantage in the number of anagrams solved (Walker et al. 2002) and also showed greater priming by weak primes (e. g., thief-wrong) than by strong primes (e.g., hot–cold), contrary to the pattern of priming observed during wakefulness (Stickgold et al. 1999). These data are consistent with a hyperassociative state of the mind during REM sleep.
Brain activity during sleep may partly explain these findings. Mesolimbic dopamine activation during sleep may influence novelty seeking and creative drive (Perogamvros and Schwartz 2012). Indeed, the dopaminergic system is thought to contribute to creativity , in particular divergent thinking, cognitive flexibility, innovative insights, and associative thinking (Chermahini and Hommel 2010; Flaherty 2005; Takeuchi et al. 2010). Further evidence comes from Parkinson’s disease patients under dopaminergic treatment, who may show increased artistic drive and productivity (Inzelberg 2013; Kulisevsky et al. 2009). Besides increased dopaminergic activity, thalamic gating of external information and deactivation of monitoring regions such as the dorsolateral PFC during (REM) sleep (Maquet et al. 1996), a structure that typically mediates volitional control of ongoing performance, may also provide additional favorable conditions for creativity, by suspending monitoring constraints on mental processes (de Manzano et al. 2010; Limb and Braun 2008). Whether increased creative drive during sleep constitutes a mechanism by which dreams may enhance creativity or facilitate the resolution of emotional conflicts remains to be addressed in future studies (Desseilles et al. 2011; Schredl 2010).
3.5 Off-Line Processing and Consolidation of Emotional Memories
Memory consolidation is an open-ended process by which new memories are progressively reorganized and incorporated into pre-existing long-term memory networks (Wang and Morris 2010). Several studies have shown that memory replay and consolidation processes occur during all sleep stages (for reviews, see Diekelmann and Born 2010; Maquet 2001; Oudiette and Paller 2013; Stickgold 2005). Both slow oscillations and sleep spindles during NREM sleep have been reported to be associated with memory (procedural and declarative) consolidation and synaptic plasticity processes (Bergmann et al. 2008; Diekelmann and Born 2010; Fogel and Smith 2011; Huber et al. 2004; Marshall et al. 2006; Rosanova and Ulrich 2005). In addition, neuroimaging studies in humans have demonstrated that NREM and REM sleep may foster lasting neural changes as well as changes in functional connectivity after perceptual, motor, or emotional learning tasks (Maquet et al. 2003; Payne and Kensinger 2010; Schwartz et al. 2002; Sterpenich et al. 2007). It is supposed that the neural traces coding for newly acquired information are reactivated during subsequent periods of both NREM sleep and/or REM sleep, thus promoting memory consolidation and reorganization (Diekelmann and Born 2010; Maquet et al. 2000; Oudiette and Paller 2013; Rasch et al. 2007; Rudoy et al. 2009). Below we report converging evidence suggesting that the activation of limbic, paralimbic, and reward systems during sleep—with or without concomitant dream experience—serves emotional regulation and memory consolidation processes.
During NREM sleep, activation of the hippocampus and ventral striatum enables the formation of a memory trace comprising contextual, emotional, and motivational components (Lansink et al. 2009). The coordinated reactivation of both the hippocampus and the ventral striatum during SWS provides a possible mechanism for the consolidation of associative memory-reward information (Fig. 3). In particular, the activation of neurons in the ventral striatum during SWS would support the selection of memories with a high storage priority (Lansink et al. 2008) and may lead to the optimization of adapted behavior during wakefulness. Consistent with this hypothesis, recent data in humans suggest that overnight consolidation of declarative memory and skill learning is guided by emotional relevance and motivational biases (Fischer and Born 2009; Sterpenich et al. 2009; Wilhelm et al. 2011).
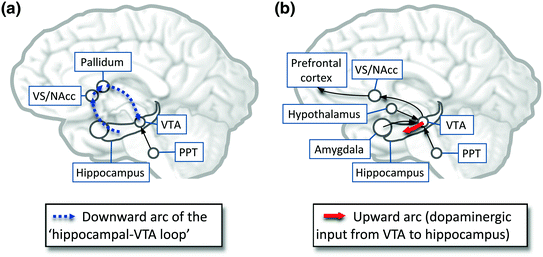
Fig. 3
Schematic illustration the reciprocal interactions between emotion /reward-related regions and hippocampal/prefrontal memory processes during NREM and REM sleep. a During NREM sleep, hippocampal activation triggers a spontaneous reactivation (replay) in ventral striatum neurons, which involves the transfer of novelty/relevance signals from the hippocampus to the VTA (thick dashed blue arrow). VTA is activated during the transition from a NREM episode to REM sleep, with induction of both tonic (hippocampus–VTA projection) and phasic (PPT–VTA) increase of dopamine . b During all REM sleep, increased bursting activity (phasic response) in the VTA may represent stimulus saliency and could fulfill reward-related functions, like stimulus-reward associations and novelty-seeking. During REM sleep, several VTA projections are activated, including the upward arc of hippocampal–VTA loop (dopaminergic input from the VTA to the hippocampus; thick plain red arrow), the NAcc, the amygdala , the orexin/hypocretin neurons, the ACC, and the PFC. All these regions have strong anatomical and functional links with the hippocampus and VTA (among others). Activation of the upward arc of the hippocampal–VTA loop contributes to synaptic plasticity and learning by enhancing long-term potentiation (Lisman and Grace 2005). PPT penduculopontine tegmental nuclei, VS/NAcc ventral striatum/nucleus accumbens , VTA ventral tegmental area . Adapted from Perogamvros and Schwartz (2012)
Active memory processing may also be consistent with sustained reward-related bursting activity of the VTA (Dahan et al. 2007) and NAcc (Lena et al. 2005) observed in rodents during REM sleep. Phasic VTA dopamine signals during REM sleep could indeed favor an off-line replay of recent emotional memory traces during this sleep stage (Walker and van der Helm 2009). These memories would then serve both as salient and novel stimuli for the penduculopontine tegmental nuclei and VTA, because recent relevant memories (e.g., emotional events, current concerns) are activated in the absence of cognitive control from dorsolateral PFC during REM sleep (Fosse et al. 2003; Schwartz 2003) (Fig. 3). This particular component of our original model still requires support from future studies (Perogamvros and Schwartz 2012).
During REM sleep , robust activation of limbic circuits is thought to promote neuronal reorganization of emotional memory traces. This reorganization involves an enhancement of the functional connectivity between the amygdala , the medial PFC, and the occipital cortex, as well as a progressive transfer of some memories along a hippocampal-cortical route (Frankland and Bontempi 2005; Sterpenich et al. 2009). Further supporting the role of REM sleep in neuronal potentiation processes, REM SD impairs the induction of hippocampal long-term potentiation in the visual cortex (Shaffery et al. 2002) and in the dorsal hippocampus (Ravassard et al. 2009) of rats. In addition, REM SD impairs hippocampal neurogenesis, which is thought to play a role in memory formation (Meerlo et al. 2009).
Taken together, these results confirm that all stages of sleep contribute to the consolidation of relevant memories involving lasting reorganization within cerebral networks.
4 Sleep Disturbance Causes Waking Emotional Dysfunctions
Early studies have shown that sleep disturbances can affect stress coping and lead to emotional and cognitive dysfunctions. In rats, suppression of REM sleep during the second and third weeks of postnatal development was found to cause anxiety, decrease in sexual activity (see also Sect. 3.3 above), and structural reductions in the cerebral cortex and brainstem weight in adulthood (Mirmiran et al. 1983). In humans, adaptation to stress was found deficient after REM SD or total SD (Kahn-Greene et al. 2007). In addition, chronic sleep disruption is associated with cognitive performance deficits (Fortier-Brochu et al. 2012; Van Dongen et al. 2003), increased aggressiveness (Kamphuis et al. 2012), and negative mood states (Zohar et al. 2005).
What may be the neurophysiological mechanisms underlying these adverse effects of sleep disturbance? In the light of recent findings on the consequences of total SD and REM SD on decision making and reward function (Gujar et al. 2011; Hanlon et al. 2010; Venkatraman et al. 2007; Yoo et al. 2007), we suggest that sleep contributes to maintaining the integrity of emotional and reward brain networks (Sects. 2.1 and 2.2). Indeed, total or partial SD impairs the normal functioning of these networks. For example, after SD, activation of the NAcc was exacerbated for risky choices in a game-like task, while insular and orbitofrontal responses to losses were reduced, denoting a diminished reactivity to punishment (Venkatraman et al. 2007). These results convincingly demonstrate that SD may disrupt competent decision making by modulating brain regions associated with risky decision making and emotional processing, such as the NAcc and the insula. Because the insular cortex is activated during both NREM (Nofzinger et al. 2002) and REM sleep (Maquet et al. 2000), one could hypothesize that SD interferes with emotional functions subserved by the insula. Moreover, decreases in D2/D3 receptor availability may account for impairments in performance, reward learning, and decision making after SD (Volkow et al. 2012). Indeed, insufficient sleep is associated with changes in reward-related decision making: people take greater risks (Harrison and Horne 2000) and are less concerned with the negative consequences of their risky behaviors (Chee and Chuah 2008; Venkatraman et al. 2007, 2011).
SD was also found to cause a failure of top-down control from the medial PFC on the amygdala . In one recent fMRI study, two groups of healthy subjects were shown 100 images ranging from emotionally neutral to increasingly aversive during a functional MRI session after one night of sleep deprivation (SD group, n = 14) or after one night of sleep (control group, n = 12) (Yoo et al. 2007). While both groups expressed similar amygdala activation levels for the neutral pictures, the sleep-deprived subjects exhibited increased amygdala response to the most negative picture stimuli compared to the control group. Importantly, a loss of functional connectivity between the medial PFC and the amygdala in the SD group was also observed. These results suggest that SD causes a reduction of prefrontal control over the limbic system, resulting in an accentuation of emotional responses to negative stimuli. SD may also lead to an amplified reactivity of reward networks in response to positive emotional stimuli (Gujar et al. 2011). More specifically, compared to a non sleep-deprived control group, sleep-deprived participants judged pleasure-evoking stimuli as more pleasant, and showed increased activation in the VTA , left putamen, amygdala and left insula. These regional increases were associated with a reduction in functional connectivity in medial and orbitofrontal cortex. Together, these studies suggest that sleep loss may impose a bidirectional affective imbalance, including increased behavioral and neural reactivity to both positive and negative emotional stimuli.
While emotional functions are influenced by processes occurring during sleep, as reviewed in the section above, the sleep/wake cycle also influences mood via two other components: the circadian (circadian phase) and homeostatic processes (duration of prior wakefulness) (Boivin et al. 1997). Even a minimal misalignment between circadian phase and sleep phase can deteriorate mood (Danilenko et al. 2003), which may also in part explain mood-related problems (e.g., irritability) in jet-lag (Waterhouse et al. 2005), and severity of unipolar depression (Hasler et al. 2010). Stress can also disrupt the circadian cycle (Meerlo et al. 2002), leading to depressive episodes in vulnerable individuals (Ehlers et al. 1988). In addition, mood deteriorates with increases in the duration of prior wakefulness (Birchler-Pedross et al. 2009; Boivin et al. 1997). The nature of the circadian-homeostatic interaction is such that moderate changes in the timing of the sleep–wake cycle may have profound effects on subsequent mood. In addition, light, with its effects on circadian and non-circadian systems, seems to play a primordial role in mood regulation (Stephenson et al. 2012).
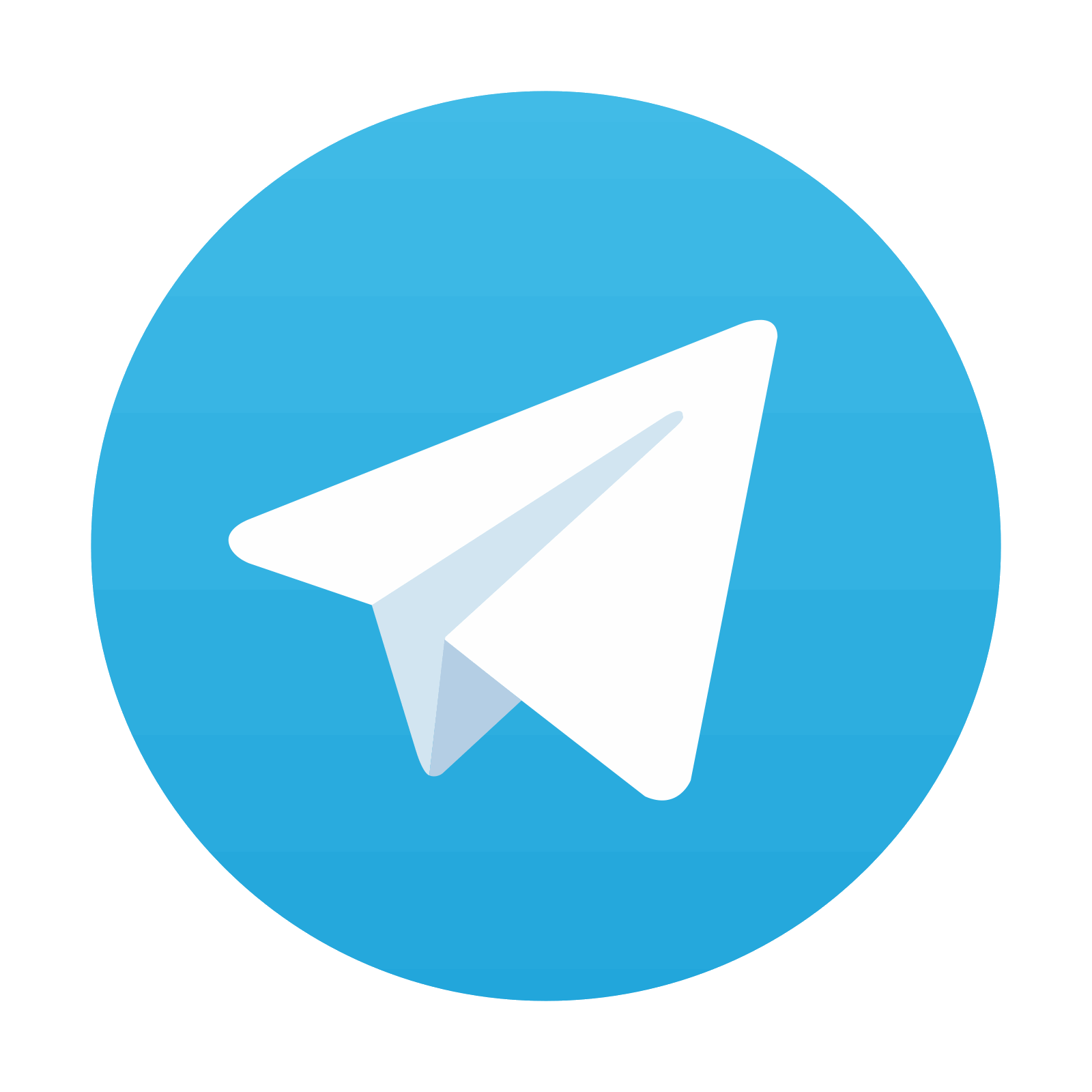
Stay updated, free articles. Join our Telegram channel
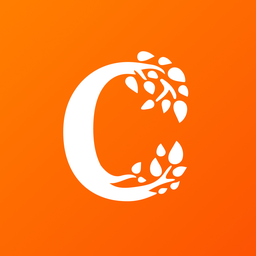
Full access? Get Clinical Tree
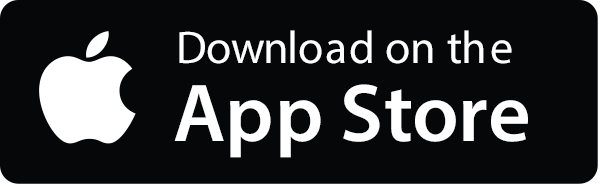
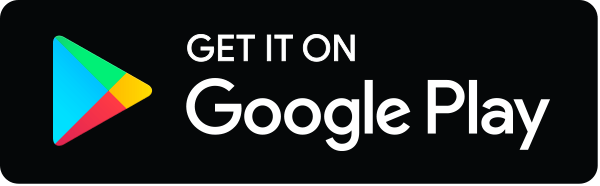