Chapter 5 Sensory and motor nerve activation
INTRODUCTION
Skeletal muscle is one of the most abundant and adaptable tissues in the human body. The heterogeneity and plasticity of mammalian muscle have become major topics of interest (Pette & Staoron 1997, Pette & Vrbová 1992). In the past two decades, the physiological mechanisms that influence the neural adaptations that accompany and contribute to these changes in skeletal muscles have been under increasing scrutiny (Gandevia 2001). They are gradually being recognized as playing a key role in evaluating the efficacy of therapeutic interventions (Enoka 1997, Pette & Vrbová 1999). This chapter outlines basic muscle and peripheral nerve physiology. Particular attention is paid to the propagation of nerve and muscle action potentials, to differing characteristics of motor units and to the concept of nerve-muscle interaction.
MOTOR NEURON TO MUSCLE ACTIVATION
NEURAL CONTROL OF MUSCLE
Smooth, coordinated movement is the output of a complex neuromuscular system. Skeletal muscle is capable of generating varying tensions and, at its very simplest, smooth, coordinated movement depends on the practical issue of contracting the required muscles in the right sequence at the right time. The control of coordinated movement is complex as different muscles combine in a variety of patterns. Appropriate combinations of excitation or inhibition of different motor neurons in dynamic series provide the required overall functional effect. Even now, there is much to understand about the way in which the neural system devises these patterns of excitation and inhibition, about the interrelationship of the afferent and efferent neural systems, and not least about how motor units are selected to achieve a particular movement and about how firing patterns are updated as the movement evolves.
THE MOTOR UNIT
The smallest unit of movement that the CNS can control is a motor unit. Defined by Sherrington in 1906, this unit consists of a motor neuron, together with its axon and dendrites, motor end plates and the muscle fibres it supplies. Motor neurons are the largest cells in the ventral horn of the spinal cord. The activity or firing frequency of these cells is dependent on their connections with afferent fibres from muscles, joints and skin, as well as their connections with other parts of the CNS.
ELECTROPHYSIOLOGICAL PROPERTIES OF NERVES AND MUSCLES
All nerve fibres have essentially the same structure and resemble a shielded electric cable. As described by Keynes & Aidley (2001), inside there is a central long conducting cylinder of cytoplasm or axoplasm surrounded by insulation: outside is another conducting layer, the electrically excitable nerve membrane. Similarly, skeletal muscle fibres are embedded in a basal lamina – a glycoprotein and collagen layer – and have an electrically excitable cell membrane (sarcolemma) that contains the intracellular fluid (sarcoplasm) and intracellular structures. Conduction of action potentials along membranes of nerves and muscles occurs because there is a potential difference between the intracellular fluid and the extracellular fluid (Fig. 5.1). The resting potential is of the order of −90mV for skeletal muscle, and −70mV for lower motor neurons. The minus sign indicates that the inside of the cell has a negative potential relative to the exterior; this potential difference can be altered by passage of ions.
GENERATION AND PROPAGATION OF ACTION POTENTIALS
Threshold
The nature of the regenerating mechanism was demonstrated both in terms of the time course of the action potential and of ionic conductance by Hodgkin & Huxley (1952). Stimulus below the threshold required to produce an action potential reduces, but does not reverse, the membrane potential. As the stimulus is increased, the potential difference across the cell membrane is reduced until it reaches the critical threshold level. At this level, the stimulus will lead to the automatic generation of an action potential. The level of the threshold varies according to a number of factors, including how many action potentials the nerve fibre has recently conducted.
After an action potential, two changes occur that make it impossible for the nerve fibre to transmit a second action potential immediately. First, inactivation (the absolute refractory period) occurs during the falling phase of the action potential. During this period, no amount of externally applied depolarization can initiate a second regenerative response. After the absolute refractory period, there is a relative refractory period during which the residual inactivation of the Na+ conductance and the relatively high K+ conductance combine to produce an increase in the threshold for action potential initiation.
STRENGTH-DURATION CURVES
The strength-duration relationship can be determined by applying single rectangular pulses of differing pulse widths to a peripheral nerve. The intensity of current required to produce a single muscle contraction (often called a twitch contraction) is recorded along with the time (pulse width) for which it is applied. Figure 5.2 illustrates the relationship between the duration of an electrical stimulus and the intensity of stimulation.
NEURONS AS CONDUCTORS OF ELECTRICITY
As a general rule, larger-diameter nerves (group Aα motor nerves) conduct impulses more rapidly and have a lower threshold of excitability than the much smaller Aδ pain fibres (Fig. 5.3). This means that threshold and motor nerve conduction velocities can be tested without exciting the pain fibres. On stimulation, larger nerve fibres also produce larger signals, their excitatory response lasts for a shorter time and they have shorter refractory periods.
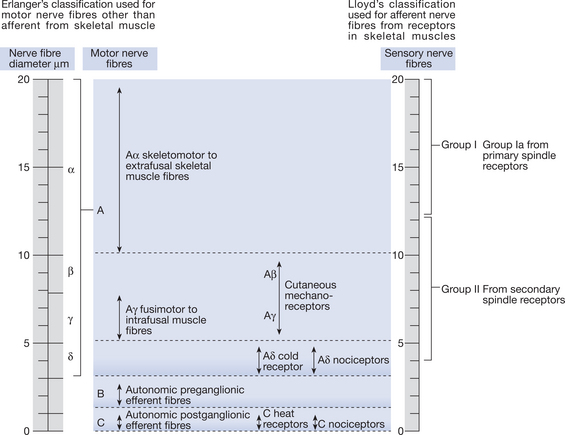
Figure 5.3 Classification of peripheral nerves according to conduction velocity and junction
(with permission from Erlanger & Gasser 1970 Human Neurophysiology, 2nd edn, Chapman and Hall).
SYNAPTIC TRANSMISSION
Acetylcholine release
When an action potential arrives at a neuromuscular junction, it causes voltage-dependent calcium ions channels in the axon terminal to open, and allows calcium ions (Ca2+) to diffuse into the intracellular fluid. Increased intracellular Ca2+ concentration causes a cascade mechanism that results in synaptic vesicles binding to the membrane in close contact with the muscle fibres. Acetylcholine released from the synaptic vesicles in the nerve terminal diffuses across the synaptic cleft in multimolar packages (or quanta) to combine with the receptor sites on the motor end plate. This alters the end-plate membrane permeability to Na+ and K+ ions and immediately depolarizes the membrane. The end-plate potential (EPP) causes a local change in potential of the muscle membrane in close contact with it. This propagates a regenerative motor unit action potential (MUAP) in all directions along the adjacent muscle membrane using the mechanism already described for the propagation of action potentials along the axon membrane. The magnitude of a single MUAP is normally sufficient to cause contraction of a muscle fibre and all fibres within the motor unit will be activated simultaneously – following the all-or-none principle.
The action of acetylcholine at the neuromuscular junction is terminated by an enzyme, acetylcholinesterase. This enzyme, embedded in the basal lamina of the synaptic cleft of the motor end plate, hydrolyses acetylcholine and thereby prevents prolonged action of the transmitter. Along the length of the muscle fibre, the muscle cell membrane (the sarcolemma) has numerous infoldings forming a system of membranes called the transverse tubular system or T tubules. As the action potential progresses along the sarcolemma, it passes close to the myofibrils down the T tubules (Figs 5.4 and 5.5).
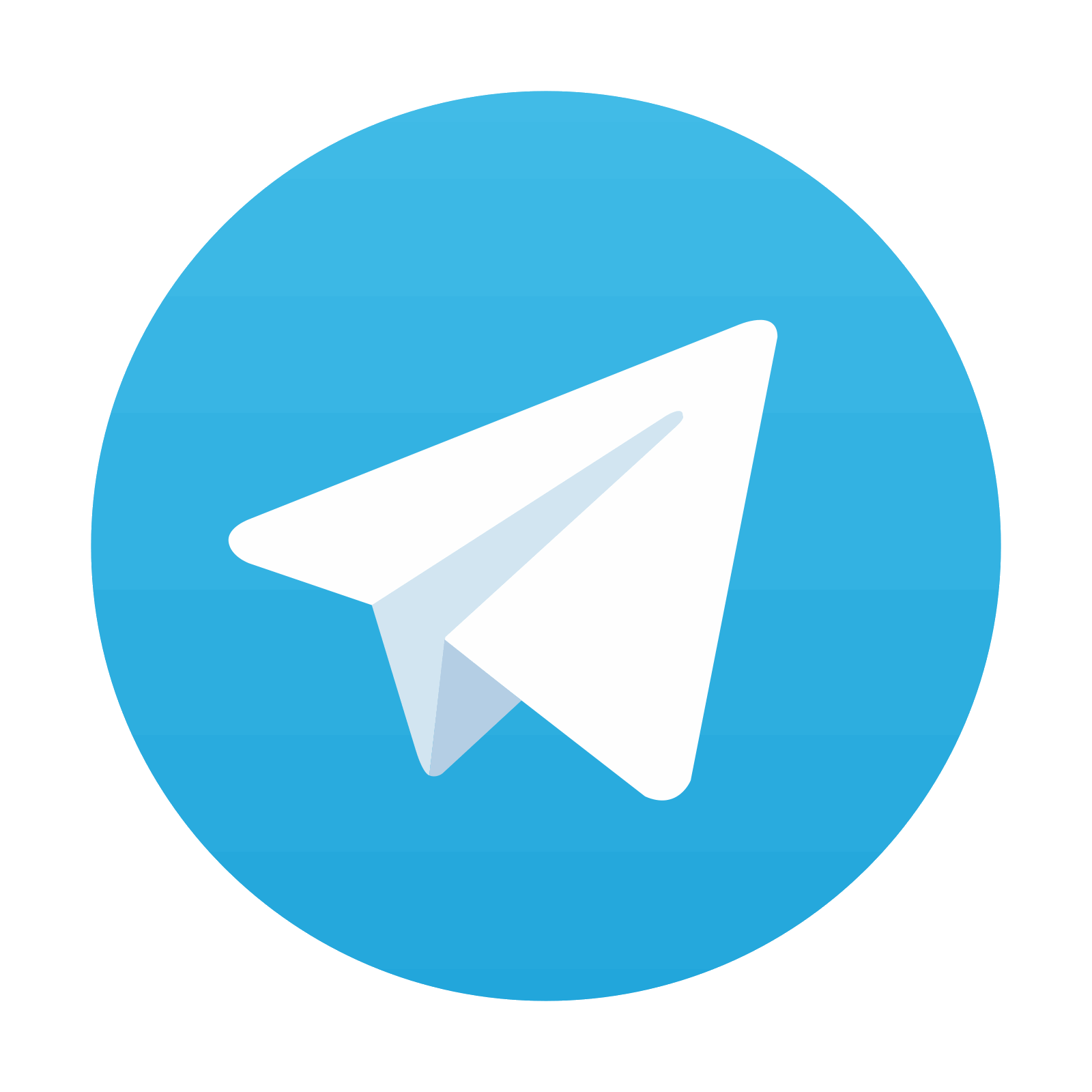
Stay updated, free articles. Join our Telegram channel
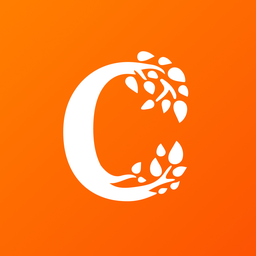
Full access? Get Clinical Tree
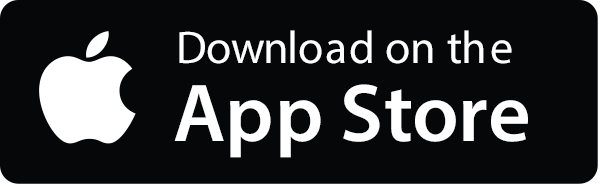
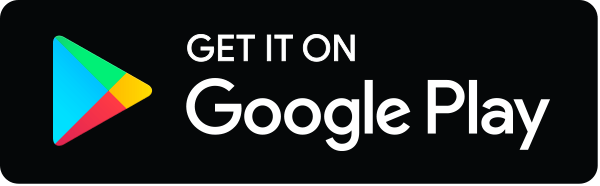