Criteria
Mild
Structural imaging
Normal
Loss of consciousness (LOC)
0–30 min
Alteration of consciousness/mental state (AOC)a
A moment up to 24 h
Posttraumatic amnesia (PTA)
0–1 day
Glasgow Coma Scale (best available score in first 24 h)
13–15
Some groups suggest normal CT scan as additional criteria for mTBI [93]. Others use the term “complicated mTBI” if any abnormality is apparent on CT scans [164]. (This concept is also used by the authors of this chapter because it implies that head injuries leading to mild symptoms may be accompanied by both normal and positive CT scans, and the latter may require distinct care.)
The origin and cause of mild TBI are not much different than that of moderate or severe cases as road traffic accidents and falls dominate the field followed by assaults and sports-/recreation-related injuries [78].
Sports-related chronic repetitive head trauma is considered as a subgroup of mTBI. Head injury is a hazard of many sports – especially combative sports such as boxing, kickboxing, soccer, ice hockey, football, and many others [37]. Concussion during sport activity is common. Concussion is a clinical syndrome with transient impairment of consciousness or disturbance of equilibrium or vision. It may be followed by post-concussion syndrome including headache, anxiety, and cognitive and psychosocial problems. Compared to the other causes of mTBI such as road traffic accidents and fall, the mechanism of sports-related head trauma is slightly different, and the intensity is lower and frequently referred to as chronic repetitive mTBI.
Pathobiology of Mild TBI
mTBI is predominantly evoked by acceleration-deceleration type of head injury, and in a majority of cases, no direct impact to the head is involved. Wide diversity of evoking forces as well as the subtle nature of structural damage makes it extremely difficult to establish relevant animal models and to understand the pathobiology. Most recent studies highlight the importance of electrolyte alterations in line with metabolic uncoupling as well as microscopic structural alterations most probably reflected in diffuse neuronal and axonal injury [69, 121, 122].
Injury Severity and Triage
As far as the severity of TBI is considered, most classifications rely on the Glasgow Coma Scale. While this approach might be well justified in severe TBI, in less severe forms, particularly mild TBI, several data indicate that a single line between GCS 12 and 13 can hardly separate moderate and mild TBI: in the case of GCS 13, the outcome is primarily defined by the neuro-worsening, seizures, and medical complications score (just like in the case of GCS 12 and 11 that are more severe forms of TBI), while in the case of GCS14 the intracranial pathology revealed by imaging will herald the outcome [30].
To this end, other factors such as loss of consciousness, posttraumatic amnesia, and their duration might help. As it has been alluded to before, most classifications require a negative CT for mild TBI; nevertheless, most recent studies identified a novel patient category that is the CT-negative, MRI-positive patient population who definitely should fall into a subgroup requiring special attention and further understanding.
Whatever classification is applied, none of them can thoroughly answer the two major questions associated with mild TBI that are: what is the actual chance for an intracranial complication in the acute setting and whether there is a possibility of late transient or permanent deficit.
Two modalities that may help in this matter are the imaging and the biological markers associated with any purported intracranial pathology (see later).
For the acute setting, the most important issue caretakers have to elucidate is the existence of an intracranial injury that may require further attention. While the majority of mild TBI cases show up at the outpatient department/emergency room without any indication of an intracranial complication, there are some conditions (“red flags”) where special cautiousness should be exercised (Table 2) [4, 142].
Table 2
Conditions (“red flags”) indicating intracranial hemorrhage in cases of complicated mild TBI
1. LOC |
2. Progressive headache |
3. Alcohol/drug intoxication |
4. Seizure |
5. Unreliable history |
6. Age under 2 years |
7. Repeated vomiting |
8. Amnesia |
9. Physical signs of skull fracture |
10. Repeated trauma |
11. Severe maxillofacial trauma |
12. Child abuse |
13. Significant subgaleal swelling/collection |
14. Coagulopathy/altered hemostasis |
15. Diabetes |
16. Age over 60 |
Unfortunately, it is rather hard to provide a clear guideline for the triage and subsequent decisions to make; nevertheless, two strategies can clearly be detected and followed: the first is close observation on the floor for 12–24 h, and the second is CT scanning of all suspicious cases (Fig. 1).
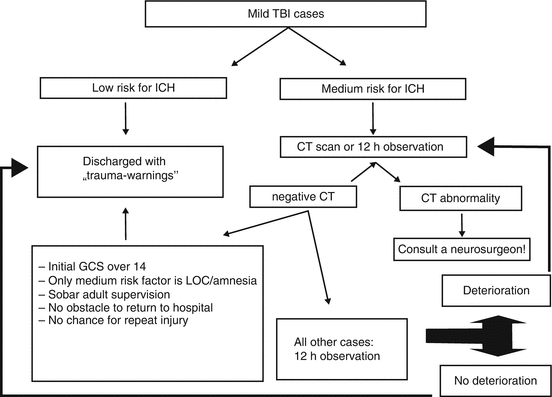
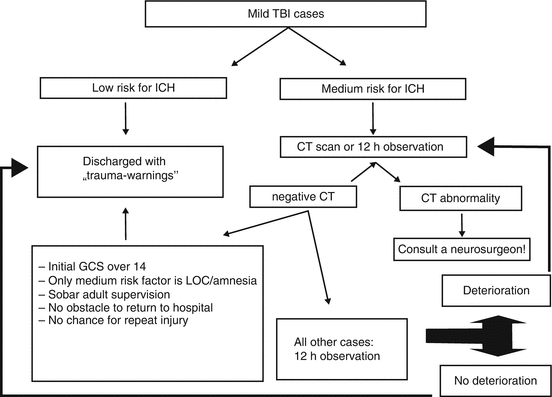
Fig. 1
Diagnostic algorithm of mTBI cases regarding risk of ICH
While cost-efficiency reports clearly support the latter strategy, negative effects of cumulative radiation over time is a major precaution. It is also obvious that not every subject can be handled the same way, as in case of young women and children observation should have priority and even if imaging is becoming necessary MRI is the primary modality to choose.
Unfortunately routine application of cognitive tests is not common, and just like imaging, cognitive tests did not prove sufficient to predict late complications either (see also sports–related injuries).
Although in mTBI the probability of associated extracranial injuries is less common, there is a significant chance for C-spine injury; thereby, precautions (in-line immobilization, C-collar) should be taken until the spine is cleared diagnostically. To exclude such injuries in a timely fashion, analysis of injury mechanisms (e.g., fall to the face) and the patients’ complaints (neck pain, numbness) may provide invaluable information.
The Role of the Neurosurgeon
Besides evacuation of intracranial bleedings complicating originally mild TBI cases, the most important task a neurosurgeon should undertake if involved in the triage or care is the identification of “red flags.” Of these, the most important is the exclusion of any factor that may be associated with altered hemostasis (Table 2). This issue has gained particular importance recently as in the aging population that represents a growing percentage of TBI cases cerebrovascular conditions frequently require the application of hemostatic treatment strategies including the K-vitamin antagonist warfarin/coumarin and drugs inhibiting platelet-aggregation such as aspirin or clopidogrel [106, 110].
When hemostasis is a problem, it is particularly important to analyze the time between injury and the first CT scan, and if any suspicion exists about a negative CT scan, it should be repeated as such second CT often times reveals the appearance of an intracranial lesion.
To this end, the role of hemoglobin-sensitive MR sequences such as hemogradient imaging or susceptibility-weighted imaging is not clarified yet although cost-efficiency issues may definitely hinder their applications (Fig. 3).
Measures to normalize altered hemostasis require individual decision with balancing between the chances for intracranial complications and their potential evolutions and cerebrovascular consequences of physiological coagulation.
It is of note that INR over the therapeutic range requires prompt intervention that can be easily executed as current evidence supports that a temporary suspension of these medications is not necessarily associated with substantially increased risk of cerebrovascular complications [124].
In case of altered hemostasis if the first CT had been performed within 4 h of injury, a considerable chance of a late-appearing ICH still exists – thereby close observation and in case of any deterioration repeat scanning are suggested.
Imaging of Mild Traumatic Brain Injury
Detection and description of the specific pathology underlying mTBI would require the development of novel diagnostic tools. These tools should be capable of (1) evaluating actual injury severity within the wide spectrum in mTBI based on objective parameters, (2) assessing prognosis, (3) avoiding “overlooked” cases, and (4) improving management of special cases as sport or combat-related trauma. The pathophysiology of mTBI is likely to involve numerous different mechanisms; however, diffuse axonal injury (DAI) is thought to play a central role, based on a large number of both human and animal investigations [120]. Although the research on mechanisms following mTBI and especially their correlation to clinical features still raises many questions, a wide range of advanced imaging methods are emerging with a promise of detecting different aspects of the traumatic sequelae.
Microstructural pathology as axonal deformation and swelling causes change in water micro-compartments and so in diffusion parameters, which can be detected by diffusion weighted and diffusion tensor imaging (DWI, DTI). Focal microscopic bleeds that may develop as part of DAI are most successfully detectable by susceptibility-weighted imaging (SWI), a method exploiting the magnetic property of iron. High-resolution, three-dimensional T1-weighted images allow precise volumetric analyses to be performed that are able to shed light on subtle changes in the brain macrostructure, due to edema and atrophy following injury. Beyond the advanced investigation of brain structure, MR spectroscopy (MRS) offers information of the metabolic state of the brain, by measuring specific magnetic signal from mainly 1H nuclei in different metabolites. Getting to higher level, the effect of injury on brain functions as perception or also cognitive tasks (as typically affected memory functions) is possible to be investigated by functional MRI (fMRI), positron emission tomography (PET), and single-photon emission computed tomography (SPECT).
Well-developed, computerized analytic methods are available to gain objective, robust, statistically relevant, and often quantitative results from most imaging modalities.
Overall, recent imaging methods provide an all-around approach to understand mTBI; as a result, several valuable “markers” have been proposed for mTBI.
However, to date the findings remain heterogeneous, and further research is needed to provide a standardized, clinically available diagnostic process.
Conventional Imaging
Computed Tomography (CT)
Computed tomography is the gold standard to examine patients with mTBI if imaging is necessary [32]. Plain cranial X-ray films were totally abandoned due to their inability to detect intracranial pathologies. X-ray gives a false feeling of confidence during the triage process while neither its’ resolution or the scope of the modality justifies this. The advantage of CT scanning is that it is readily available in every trauma center, and even agitated patients can be examined due to the short acquisition time. It provides voxelwise X-ray attenuation maps with good spatial resolution, and acute hematomas can be easily visualized due to their high degree of X-ray attenuation.
The disadvantage of the technique is that it applies ionization radiation thus it may add a risk to cancer development – a problem significantly reduced in novel low radiation instruments. Other disadvantage is that diffuse white matter lesions may remain hidden on the images.
The main purpose of CT scanning is the identification of those patients who need hospitalization and further observation. It should be noted that only a minority of patients (<10 %) with inclusion criteria for mTBI will have positive CT scan [40, 55, 145]. Overwhelming majority of classifications (vide supra) then declare these patients as complicated or moderate TBI cases.
Several guidelines (e.g., [61, 63]) have been put forward to determine the circumstances when CT scanning is required. The common points for indication of a CT in patients with mTBI are the following: loss of consciousness, vomiting, amnesia, suspected cranial fracture (basal, open, or depressed), neurological deficit, coagulopathy, post traumatic seizure, age > 60 years, and high energy injury (i.e., fall from height, motor vehicle accident, etc.) (also see Table 2).
By several definitions, traumatic brain injury is considered “mild” when the CT is negative (vide supra). Thereby in this chapter, we allude to the use of CT in cases of suspected mTBI.
In suspected mTBI, CT may visualize small macroscopic hemorrhages such as cerebral contusions, traumatic subarachnoidal hemorrhages, and extra- or subdural hematomas. Nevertheless, small hematomas in patients with mTBI may remain hidden due partial volume effect (mostly on the bone-brain interface) on the temporal and frontal skull bases. Such hemorrhages in mTBI appear to progress within the first 2 h after injury and reach their final volume within 24 h [58]. It is of note that pathology on acute CT in mTBI may not predict the outcome 3 months after injury [82], and clinical variables together with age may be stronger predictors for long-term outcome [62].
The above-listed limitations of CT in terms of resolution and/or outcome prediction ignited the search for advanced neuroimaging modalities.
Conventional MRI
Conventional MRI (T1- and T2-weighted, fluid attenuation recovery, FLAIR) is more sensitive to focal injuries than CT; higher number and volume of lesions are detectable [67]. However, in many cases of mTBI, conventional MRI also fails to detect the diffuse and subtle damage, and the clinical interpretation of detected lesions is debatable [59].
Although CT remains the gold standard imaging modality for TBI, MRI is suggested to be performed in children and young women due to the absence of ionizing radiation. Unfortunately MRI has some contraindications to be kept in mind, as a patient with pacemaker, claustrophobia (open magnet can be an alternative), metallic foreign body, etc.
Advanced MRI Techniques
Diffusion Tensor Imaging (DTI)
DTI measures Brownian movement of water molecules and applies at least six diffusion gradient directions, thus is able to provide information on the extent and directionality of diffusion. Fractional anisotropy (FA) refers to the degree of directionality, calculated from the variation in the eigenvalues of the diffusion tensor, while mean diffusivity (MD) or the synonym apparent diffusion coefficient (ADC) refers to the overall, rotationally indifferent mobility of water molecules [7]. The character of diffusivity in brain is widely accepted to be associated with fiber tracts, i.e., axons and myelin sheath. Thus, FA and MD are theoretically able to detect DAI, an effect of shear-strain deformation of the fiber structure, regarded to lie behind mTBI. Furthermore, the sensitivity to changes of water micro-compartments makes DTI able to assess different subtypes of edema and other important components of the traumatic tissue [10].
A large cohort of studies investigated diffusion in mTBI focusing on several different relations, e.g., with age, acute or chronic phase, clinical symptoms or neuropsychology tests, recovery, and sports- and combat-related injuries.
Many investigations on mTBI found reduced FA or elevated MD (ADC) in mildly injured patients (Fig. 2) and often interpreted the findings as reduced integrity, i.e., misalignment of axonal and myelin structures due to shear-strain forces, including local expansion of axonal cylinder or axonal disconnection [3]. On the other hand, other studies observed elevated FA or reduced MD acutely after mild injury along several white matter regions [94]. One possible underlying mechanism is cytotoxic edema, as in this condition the injury induced altered function of gated ion channels results in intracellular swelling and decrease in extracellular water that causes reduced radial diffusivity. The output yielded by DTI may show a summarized effect of the two basic mechanisms, microstructural disintegration and cytotoxic edema. A recent study also found bidirectional irregularities in DTI parameters after injury [86]. The actual dominance of these substantial mechanisms in the white matter may theoretically depend on temporal, spatial factors, attributes of the patient, and the circumstances of injury. Future research should shed light on the proper interpretation of the different diffusion indices possibly by focusing also on less robust parameters such as eigenvectors and eigenvalues.
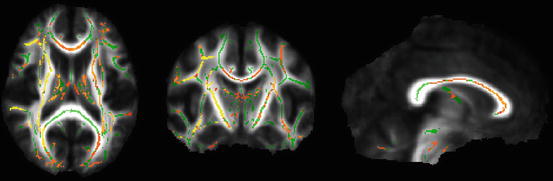
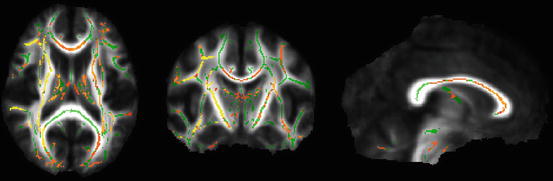
Fig. 2
This voxelwise analysis (Tract-Based Spatial Statistics, FSL – FMRIB’s Software Library) compares an mTBI group’s acute data and a matched control group (n = 14 in both groups, respectively). The red-yellow voxels represent significantly (corrected p < 0.05) decreased fractional anisotropy (FA) in the mTBI group compared to the control group. Significant voxels (yellow-red) are overlaid on the group mean white matter “skeleton” (green) and the group mean FA image (grayscale). Images are shown in radiological convention (right = subject’s left)
Findings of follow-up studies are also various; some longitudinal studies revealed partial normalization of DTI indices after different periods, while other investigations indicated traumatic microstructural alteration to be more stable or even to evolve. There are promising observations of the relation of DTI findings with cognitive or psychological dysfunction and clinical outcome, especially in moderate to severe cases [109].
An advanced analysis of DTI data, DTI tractography, was recently shown to be competent in assessing mTBI and outcome prediction.
Diffusion spectrum imaging (DSI) that is thought to resolve crossing fibers (unlike conventional DTI) recently provided novel insights of the human white matter microstructure [161]. This altogether with novel connectomic techniques may revolutionize understanding microstructure alteration in mTBI.
Volumetric Analysis
Due to the high resolution and contrast of T1-weighted images, especially the three-dimensional T1 images (e.g., MPRAGE), it is possible to closely estimate volumes of specific brain structures. First, this was achieved by manual measurements, which were later replaced by automatic segmentation algorithms, voxel-based morphometry techniques, and other computer-based methods offering the estimation of both global and regional brain volumes.
Different manifestations of brain atrophy following injury were identified in a large number of morphometric studies conducted on mixed (mainly moderate to severe) TBI populations [163]; injury severity or cognitive function was correlated with atrophy rate; in one group outcome was found to be independent from atrophy; the association of post- traumatic stress disorder with atrophy of whole brain, corpus callosum, anterior cingulum, and hippocampus was presented by some studies.
However, a low number of studies investigated homogenous mTBI groups. MacKenzie et al. found global atrophy developing in 3 months in a group of mild and moderate injured patients that was correlated with LOC [91]. Specific gray matter volume decrease was also shown, but was not predictive for outcome. A recent follow-up study raised the possibility of post-injury edema formation in mTBI that is detectable by volumetric analysis [157].
Susceptibility-Weighted Imaging (SWI)
SWI is particularly sensitive in detecting both intravascular venous deoxygenated blood and extravascular blood products [52].
It was shown to be the most sensitive modality in detecting microhemorrhage, primarily in pediatric TBI of mixed severity [155]. The correlation of SWI lesion number, volume, and location with neuropsychological functioning or with outcome was also presented in children.
In contrast, adult data and especially studies of mTBI are limited. A study proved the superiority of SWI over CT and conventional MRI in sensitivity to microhemorrhage in a group of adults with dominantly severe TBI patients [1].
Microhemorrhages do not seem to be frequent in mTBI. Based on data from amateur and professional boxers and on experiences of SWI using diagnostic centers, SWI lesions in mTBI occur in about one out of ten patients. This lesion occurrence is comparable to the lesion occurrence (not only microhemorrhages) detectable with conventional imaging methods. Future research should reveal the relation between injury circumstances, conventional imaging, SWI, and outcome, also providing data on the overall clinical importance of SWI in lesion detection in mTBI (Fig. 3).
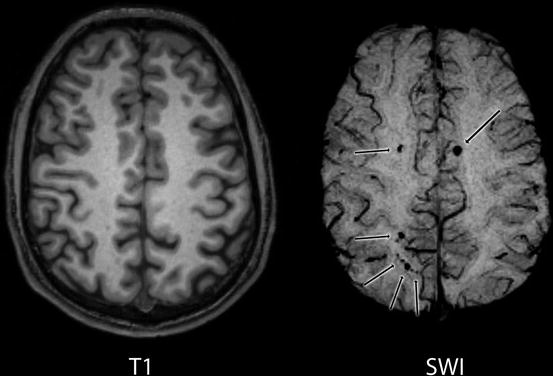
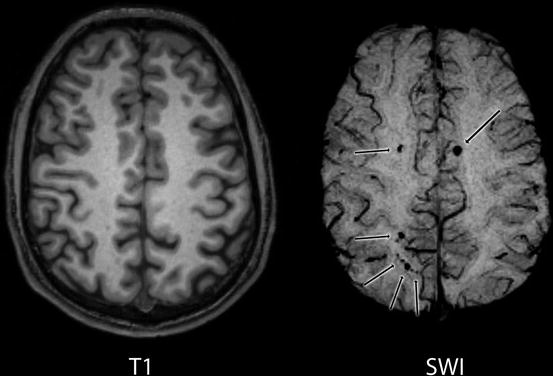
Fig. 3
T1-weighted and SWI images of a mTBI patient (GCS = 14, LOC = 3 min. PTA = 2 h) acquired 20 h after injury. SWI revealed several microbleeds (black arrows). These lesions are scarcely, or not detectable on the T1 image. CT image was diagnosed without trauma-related pathology
Magnetic Resonance Spectroscopy (MRS)
By measuring solute-specific magnetic signals from 1H nuclei, MRS offers in vivo chemical information of the brain tissue. This method can be used to detect the potentially altered metabolism following mTBI.
The main peaks of a proton MRS spectrum refer to the following metabolites: N-acetylaspartate, marker of neuronal integrity; choline which may be altered in inflammation, proliferation, or membrane damage; myo-inositol, which is a glial marker; lactate, that is elevated in ischemic/hypoxic conditions; creatine and phosphocreatine, which are related to energy metabolism but are often assumed to be relatively constant, so are widely used to standardize other metabolites; and glutamate and glutamine (glx when combined) which are important neurotransmitters or metabolites.
First MRS studies on mTBI patients showed various irregularities in metabolites: decreases of N-acetylaspartate (NAA) were found in the corpus callosum and parietal white matter [49]; another study found normal NAA levels but increased choline levels in the frontal lobe [44]; a finding also described in the occipital lobe [49]. A 2-month follow-up investigation presented recovery in NAA in pericontusional tissue.
However, correlation of metabolite irregularities and clinical features were not presented convincingly, only in severe TBI patients. The instability of creatinine levels in mTBI was suggested to be a confound factor as creatinine was suggested to change in hypo- or hypermetabolic state or different diseases.
Later studies conducted on more homogenous mTBI groups with better-defined acquisition time points supported different metabolites (mainly NAA and choline) to be associated with outcome [159].
Interestingly, relation between NAA reduction at certain white matter regions and posttraumatic headache was also postulated.
To conclude, MRS has a great potential in elucidating the effects of mild injuries on the brain. By taking acquisition circumstances to common denominator and revealing the specificity of the different metabolic alterations, MRS may become a helpful diagnostic and prognostic tool.
Functional Magnetic Resonance Imaging (fMRI)
Functional MRI detects local hemodynamic changes following increased metabolic rate in neural activity, by measuring the blood oxygen level dependent (BOLD) contrast.
Specific cognitive, motor, and memory tasks or sensory stimulation is repeated, and the associated BOLD signals are compared. Functional connectivity investigation reveals brain areas with correlated fluctuations (i.e., coupled functionality) during an experimental task or resting state (in the absence of any active task or external stimulus).
There is a growing body of evidence that fMRI is able to detect altered patterns of brain activation following mTBI [64].
Most studies concentrated on memory functions, especially working memory, given that cognitive impairment is a major concern in mTBI. The altered activation that is primarily detected in the dorsolateral prefrontal cortex was suggested to underlie working memory dysfunctions. A few studies focused on spatial working memory or declarative memory. Non-memory function was also tested, for instance, by a motor-sequencing task.
In these studies, various injury-related changes of BOLD signal level and distribution were detected. Some studies also found attenuated activation in mTBI patients (Fig. 4) that may be a result of injured neural network. Others reported increased or additional activation. These findings may be explained as results of neural reorganization or functional accommodation [64].
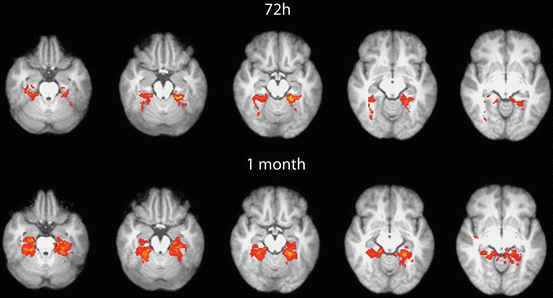
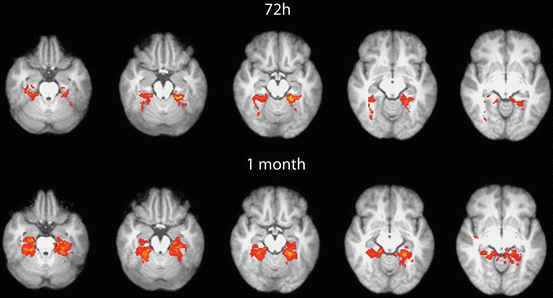
Fig. 4
Decreased level and extent of activation was detected during spatio-visual memory task (Roland’s Hometown walking) in parahippocampal regions by fMRI at 72 h compared to 1 month after injury within a group of mTBI patients. The red-yellow voxels show the significant group activations (10 patients, same Z score threshold) that are overlaid on MNI 152 standard brain slices. This activation difference was validated by statistical analysis as well (paired t-test)
Correlation between BOLD signal change and neuropsychological findings or task performance was proposed; however, the alteration of BOLD signal distribution was observed independent of clinical signs as well. A relatively low number of longitudinal studies suggested that the cessation of symptoms over time is associated with the normalization of cortical patterns.
The recent wave of resting state fMRI studies on mTBI patients provided further important insights to the functionality of the injured brain. Depending on explored areas, both decreased and increased connectivity was registered. Alterations in the default mode network connectivity are suggested to have clinical connotation [68].
Integration of structural and functional connectivity data may be a subsequent step to understand the background of mTBI related complaints [135].
Nuclear Imaging: Positron Emission Tomography (PET) and Single-Photon Emission Computed Tomography (SPECT)
PET
PET imaging is a robust method to reveal regional glucose metabolism of the human brain, generally using isotopes as [18F] fluorodeoxyglucose (FDG). However, it has been less widely used as fMRI because of its lower availability, spatial-temporal resolution, and ionizing radiation, especially in the investigation of the healthy brain.
In severe TBI, a triphasic metabolic pattern was demonstrated both in humans and animal models, which involved an acute hyperglycolysis followed by a prolonged period of metabolic depression and a final recovery [12]. Data in mTBI are limited and inconsistent. Studies agree that metabolic irregularities may persist for months or even years after injury, but both hypo- and hypermetabolism were detected along different brain areas [26].
SPECT
Other possible way of assessing brain metabolism is the measuring of blood flow by SPECT. This method is more accessible and cheaper than PET; thus, broader range of information was obtained in mTBI.
Studies that focused on the acute phase of mTBI revealed hypoperfusion in various brain regions including the frontal lobes [5]. The incremental validity of SPECT beyond CT was also established; however, quantitative correlations to clinical aspects were not supported. Patients with persistent posttraumatic complaints also show hypoperfusion, often in the frontal and temporal lobes. Although the clinical significance of SPECT irregularities is not clear yet, this method was proven to be highly sensitive to mTBI; thus, a normal post-injury SPECT scan may potentially exclude unfavorable outcome [50].
Biomarkers of Mild Traumatic Brain Injury
Despite a substantial improvement in this area, mild TBIs, compared to moderate and severe brain injuries, remain often challenging to diagnose, mostly due to rapid resolution of acute signs and symptoms and the absence of objective evidence of injury on neuroimaging.
Furthermore, Ruff and colleagues [128] conducted an accurate analysis on potential confounders in the current diagnostic schemes for TBI and highlighted the difficulty in differentiating mild TBI from non-TBI pathologies that may present with similar symptomatology. Such distinction is particularly relevant in light of the high reported incidence of mild blast TBI in military personnel returning from combat [85].
The recognition of the impact of previously undiagnosed TBI further pointed out the urgent need for accurate and objective diagnostic criteria that can be represented by the clinical use of biomarkers. Indeed, a consensus definition of TBI [100] recognized that advanced imaging and other novel biomarkers might allow better characterization of TBI in individuals, advancing care to personalized management. This could be a pragmatic solution to the problem of diagnosis and categorization allowing establishment of effective clinical protocols and pathways for clinical care.
Importantly, although most patients with minor head injury can be discharged without sequelae after in-hospital observation, a small proportion of patients have intracranial injuries (ICI) and require neurosurgical intervention [55]. Early diagnosis of ICI by computed tomography (CT) followed by early surgery is very important in the treatment of such patients. Although, several rules have been published aimed at identifying patients with higher risks for CT findings and/or neurosurgical intervention, the use of CT for minor head injury has become increasingly common due to several causes. A large proportion of mTBI patients, between 30 and 50 %, are intoxicated [114], which confound several of the clinical predictors included in the decision rules. In addition, approximately 10 % of physicians reported that New Orleans Criteria guidelines and Canadian CT Head Rule were uncomfortable to apply, and the guidelines have been shown to be misinterpreted by approximately 5 % of physicians [144].
More selective use of CT scans for investigation of patients with minor head injury could lead to large reductions exposure to potentially harmful ionizing radiation [138] as well as health-care costs. The challenges of early diagnosis and identification of disease-modifying drugs have created a need for biomarkers that reflect core elements of the disease process. The possibility of using new biomarkers in patients with mTBI could provide a rapid, definitive, and cost-effective diagnostic test for brain injury that prove invaluable in diagnosis and improve management of brain-injured patients reducing significantly CT usage.
Several studies from different research groups have explored the ability of different biomarkers to aid in decision making in the management of patients with mild TBI. These efforts initially concentrated on S100, but lately new biomarkers have been explored.
Here, we review the candidate serum biomarkers for mTBI. We focus on established biomarkers (biomarkers evaluated in several studies by various research groups) as well as novel promising markers, discussing their potential roles as TBI diagnostic tools and providing a practical guide to their implementation in the clinic.
Biochemical Markers of Brain Damage for mTBI
A biomarker is an objective measure of a specific biological or pathological process that can be measured in body fluids such as cerebral spinal fluid (CSF) and blood. Specifically, traumatic injury to the brain causes cellular damage and disintegration, leading to release of cell type-specific proteins such as S100B, glial fibrillary astrocytic protein (GFAP), and ubiquitin C-terminal hydrolase (UCH-L1) into biofluids (CSF and blood). As a result, biomarker concentrations accurately reflect cerebral pathological changes and are directly proportional to the magnitude of the injury.
The blood, easily and routinely accessible following mild TBI, is the optimal source of biomarkers, and the presence and quantity of the biomarker can be accurately determined by sensitive enzyme-linked immunosorbent assays (ELISAs).
Specific brain injury biomarkers can have utility as blood-based TBI diagnostic and monitoring tools. The use of biomarkers would be of particular value in identifying mTBI patients with ambiguous neurological symptoms or intoxicated or when neuroimaging cannot be obtained or is non-diagnostic. In addition, biomarkers for TBI could have important prognostic functions. Accurate identification of patients who sustain mild TBIs will facilitate development of guidelines for return to work or sports activities and also provide opportunities for a better management and pathways for clinical care.
S100B
The S100B is a calcium-binding protein mainly expressed in astrocytes but also present in other nervous cell types such as microglia and neurons and extracerebral cells such as adipocytes, chondrocytes, and bone marrow cells [36]. A number of studies have shown that levels of protein S100B in biological fluids correlate with the presence and severity of neurological disorders. In 1995, Ingebrigtsen et al. [60] reported, for the first time, the use of serum S100B in patients with mild TBI. They found that S100 levels were associated with the development of symptoms in 67 % of patients with negative CT after minor head injury. Since this original study, several studies from different research groups have explored the ability of this biomarker to aid in medical decision making in the management of patients with mTBI. Although few researches tried to assess the relationships between S100 levels and short- and long-term symptoms with contrasting results [61, 130], significant efforts have been concentrated on the diagnostic accuracy of S100B for intracranial injury evident by CT. Consistently, S100B demonstrated high sensitivity (75–100 %) for CT-evident injury [158] but low specificity due to the extracerebral sources of the protein [126].
Recently, Unden and colleagues [158] conducted a meta-analysis involving 2,466 adult mTBI patients with the aim of evaluating whether S100B can predict normal CT findings after minor head injury. They concluded that low serum S100B levels (<0.10 μg/L) taken within 3 h of injury predict normal CT findings after mTBI in adults with negative predictive values of 90–100 % [158].
Despite these very promising results, as mentioned above, a significant limitation to the use of S100B as a potential screening agent for brain injury is the lack of specificity in the setting of trauma patients [126]. Nevertheless, the inclusion of S100B in a panel of brain biomarkers can improve sensitivity and the predictive value of the initial CT scan.
Evidence for Novel Candidate Biomarkers in Mild TBI
S100B is the most commonly studied and more frequently used biomarker. However, the lack of specificity for the brain has been limiting its application as diagnostic tool in brain injury. As a result, other more specific biomarkers have been investigated to obtain a clinically useful specificity and positive predictive value (PPV) for brain injury after mild TBI. These candidate biomarkers include glial fibrillary acidic protein, neuron-specific enolase, and ubiquitin C-terminal hydrolase. Of these, glial fibrillary acidic protein and UCH-L1 seem most promising because of the clinical specificity.
The following sections review our current knowledge on these novel protein biomarkers with respect to their diagnostic and prognostic ability in mild head injury. However, these markers have not been sufficiently investigated in mTBI, and further studies are needed before any conclusions can be drawn.
Neuron-Specific Enolase
Neuron-specific enolase (NSE) is a glycolytic pathway enzyme, localized predominantly in neuronal cytoplasm. This protein, originally identified in neurons, was successively found in other cell types including erythrocytes, platelets neuroendocrine cells, and oligodendrocytes.
Several studies demonstrated that NSE is a sensitive indicator of neuronal cell death and serum levels increase after TBI and correlate with the severity of the injury [125, 165]. To date, there are still little data on the utility of serum NSE measurements in mild head-injured patients. However, NSE concentrations have been shown to be higher in patients with mild TBI compared to control [35]; there was no convincing evidence of ability of this marker to function as a stand-alone predictor of post-concussion syndrome (PCS) [35].
In a recent study of 141 patients with mTBI evaluating the predictive value with respect to the short- and long-term neuropsychological outcome, NSE concentrations within 4 h after injury alone had a limited discriminative power [156]. Conversely, NSE in conjunction with other clinical and demographic variables demonstrated high accuracy (c-statistic 0.895) in predicting poor outcome at 6 weeks post-injury [156].
Glial Fibrillary Acidic Protein
Glial fibrillary acidic protein (GFAP) is a monomeric intermediate filament protein that represents the major component of the astroglial cytoskeleton [39]. It is found in glial cells in gray and white brain matter [103] and is highly specific marker for CNS pathology as is not found outside the central nervous system.
In studies concerning severe TBI, the relation between injury severity and serum GFAP has been investigated and assessed. Levels of GFAP are related to the severity of brain injury and outcome [105, 160].
Recently, two concurrent studies examined the value of serum GFAP as a marker of brain injury in patients with mild TBI [101, 112]. In 108 patients with mild and moderate head injury (GCS scores of 9–15), the accuracy for intracranial injury evident by CT scan was 0.79, with a sensitivity of 97 %, a specificity level of 18 %, and the negative predictive value of 94 % using cutoff level of 0.035 ng/mL. Further more, with a GFAP cutoff value of 0.17 ng/mL, the patients requiring neurosurgical intervention findings were identified with a sensitivity level of 100 % and a specificity level of 42 % and negative predictive value of 100 % [112]. Accordingly, in their study including 94 patients with mild brain injury (GCS between 13 and 15), Metting et al. [101] reported higher GFAP levels within 3 h after injury in patients with an abnormal admission CT compared to patients with a normal CT. GFAP showed a high negative predictive value for MRI 3 months post-injury (0.82) indicating that low GFAP levels in serum predict a normal MRI. When compared to S100B, GFAP appears a more sensitive marker. GFAP was found to be related to imaging and outcome as defined by return to work, whereas S100B was found not to be of prognostic value in this patient category. However, the authors conclude that as the category of mild TBI includes less severe injuries with no detectable GFAP (63 % in their study), the predictive value of GFAP appears limited and not useful in making clinical decisions or for prediction of outcome in this patient group [101].
Ubiquitin C-Terminal Hydrolase
Ubiquitin C-terminal hydrolase (UCH-L1) is a highly abundant neuron-specific enzyme, representing between 1 and 5 % of total soluble brain protein [84]. It has been suggested that UCH-L1 plays an important role in the removal of excessive, oxidized, or misfolded proteins during both normal and neuropathological conditions including neurodegenerative disorders [75]. This protein is released into the extracellular space as a consequence of cell destruction under pathological conditions. Measurable amounts of this damage marker are present in cerebrospinal fluid (CSF) and blood and are directly proportional to the magnitude of the injury. Because of these characteristics and its high brain specificity and abundance in the CNS, UCH-L1 has been proposed as a novel biomarker for TBI. Previous experimental and clinical studies have shown significantly increased UCH-L1 levels in CSF and in serum in severe TBI patients compared to uninjured subjects. Also an association between UCH-L1 concentrations and injury severity and clinical outcome was observed [104].
A study has been conducted recently investigating UCH-L1 in 96 adults with mild and moderate TBI (GCS score 9–15) [113]. Mean serum UCH-L1 levels were significantly higher in patients with CT positive (1.618 ng/ml) compared to patients with CT negative (0.620 ng/ml). Using a cutoff level of 0.09 ng/mL, the sensitivity and specificity of UCH-L1 for detecting intracranial lesions on CT were 100 % and 21 %, respectively, and a negative predictive value of 100 %. In addition, a correlation between UCH-L1 concentrations and measures of injury severity including the GCS score and neurosurgical intervention was observed.
Evidence for Biomarker Use in Children with Mild Head Injury
Traumatic brain injury (TBI) is a leading cause of morbidity and mortality in infants and children and remains a public health problem of enormous magnitude. Case series from multiple trauma centers report that 75–97 % of trauma deaths in children result from head injuries [154]. The incidence of TBI is calculated as 1/285 in babies younger than 1 year [65], and up to 80 % of deaths in children younger than 2 years are due to inflicted TBI – often referred to as shaken baby syndrome [14, 77].
Patients with severe TBI are easy to recognize in clinical practice, but the correct diagnosis of mild TBI (mTBI) in babies and younger children is difficult even for experienced physicians, partly because questioning rarely provides a good history of trauma and partly because infants present common nonspecific clinical symptoms [21] with an unremarkable physical examination [54, 107].
Despite algorithms for the evaluation and triage of children and adolescents with mTBI are available [79] to reduce unnecessary CT scan and inpatient observation, most CT and short hospitalization can still be potentially avoided, because 93 % of children with mTBI have no intracerebral lesions [57].
Several reports suggested that biomarkers might be valuable in pediatric TBI [15, 77], and there is a clear trend toward increased international efforts to evaluate the use of biomarkers in pediatric TBI [23, 77].
However, while the adult literature related to biomarkers in TBI and outcome prediction is extensive, the pediatric literature is still limited, especially for mTBI. In addition, the biomarkers which are best studied in adults have pediatric-specific limitations; NSE is affected by hemolysis, a particularly important problem in infants and young children [13], while S100B has high normative concentrations in young children [19].
In a study evaluating 100 patients after iTBI and nTBI, the sensitivity and specificity of the initial NSE for identifying TBI were 71 and 64 %, respectively [14]. Serial serum sampling of NSE in children (n = 152) with mild (61 %) and severe (30 %) TBI shows that higher concentrations are associated with worse outcome. The initial and peak NSE concentrations showed a stronger correlation with outcome in children less than 4 years than those >4 years of age [16].
The role of the S100B marker in the initial assessment of children with mTBI is still controversial, and not really defined [42]. Berger and colleagues report [17] that, in children under 1 year of age arriving at the emergency department with nonspecific symptoms but with suspicion of TBI, S100B was neither sensitive nor specific for iTBI, and an increased level was measured in 90 % of patients with non-brain injury. However, the same group showed in a later study that the mean concentrations of S100B were significantly greater than normal control concentrations in children with mild and severe TBI (n = 152), and there was a significant correlation between outcome and serum marker in children both over and under 4 years [16]. Bechtel et al. [11] reported a study with S100B levels in serum after closed head injury in children. They found a PPV for intracranial injury of only 20 % but a high negative predictive value of 90 % at the same cutoff. They also investigated the impact of bone fractures on S100B levels and found that these extracranial injuries may be responsible for the poor specificity of S100B in trauma. In a study of 109 children, Castellani et al. [23] showed that S100B gives a sensitivity of 100 % and reduces unnecessary cranial CT scans. Hallen et al., who did a study on 111 children, suggested that S100B could be a valuable diagnostic tool added to those used in clinical practice today [53]. Recently, Bouvier et al. [21] evaluated the utility of serum S100B measurement in mTBI management on a large cohort (n = 446) of children with closed head trauma divided into three groups on the basis of TBI severity (minimal, mild, and severe). Levels of S100B in serum correlated with severity of injury. In addition, measurement of S100B accurately identified patients with CT positive (100 % sensitivity and 33 % specificity) and children with poor clinical evolution (100 % sensitivity and 36 % specificity), demonstrating a potential clinical utility of S100B for the management and outcome prediction of children with mTBI.
Recently, two novel candidate biomarkers, UCH-L1 and αII-spectrin breakdown product 145 kDa (SBDP145), have been evaluated by Berger and colleagues [18] in children with TBI and healthy controls. Serum UCH-L1 and SBDP145 concentrations were significantly greater in TBI patients than in controls, but the increase was exclusively seen in subjects with moderate and severe TBI, no after mild TBI. Both markers were correlated with glasgow outcome scale (GOS), and this correlation was stronger than the correlations with NSE, S100B, or MBP [18]. These encouraging results support and highlighted the need for further studies with more inclusion.
Venous cannulation is not always performed in children with mTBI, especially in asymptomatic subjects. Urine or capillary measurements of biomarkers would be a suitable option because this sampling method is less invasive and can be easily used for children in the emergency department. Nevertheless, separate reference and injury values must be studied before this method can be considered [158].
Taken all together, these data suggest that after validation in a multicenter study that includes a high number of cases, biomarker determination might be an invaluable diagnostic tool that could avoid unnecessary irradiation minimizing potential health risks [138], produce hospital cost savings, and reduce the duration of mTBI management in pediatric emergency departments.
TBI in the Military
Based upon the data from a population-based survey of service members and veterans who served in Afghanistan or Iraq, the RAND Corporation estimated that 19.5 % of the previously deployed persons suffered from a “probable TBI” for a total of 320,000 head injury [66]. Between the years of 2005 through 2009 (http://www.health.mil/Research/TBI_Numbers.aspx), a sharp increase in the number of TBI diagnosis, mainly cases of mild TBI, has been reported.
The increased awareness and recognition of the impact of TBI in the military population spurred the interest of US military in a method to objectively determine brain injury and in the development of a point of care test for TBI.
However, in addition to the complexity of TBI, the peculiar characteristics of military setting create another layer of difficulty and complexity in the ability to diagnose TBI [131].
Civilian and the military environments are significantly different. In a civilian setting, following a head trauma, a subject can be quickly transported to the nearest emergency department, where can be promptly evaluated by an emergency physician and undergo a CT or an MRI scan, if is necessary. Conversely, the battlefield is often a very austere environment. In the military setting, the head trauma can occur in remote locations either before, during, or after some type of battle and possibly lasting hours before casualties can be clinically evaluated [131]. Furthermore, evacuation priorities and logistics may impact the ability to evaluate suspected mTBI cases as the ability to image the brain is only located at specific locations in the theater of operations.
Although there is the list of tools to screen casualties such as the Military Acute Concussion Evaluation (MACE) that is well integrated into the military medical evaluation [102], they still rely on self-reporting, may be affected by confounders such stress or fatigue, and may show low sensitivity when administered greater than 12 h [28].
The ideal method for the diagnosis of TBI would be a rapid, definitive, and cost-effective blood-based diagnostic test for TBI. Having such a tool would assist military medics to objectively and quantifiably diagnose the presence and severity of TBI and therefore better provide for the casualty’s and assist in making important operational decisions such as allocation of resources and determining when the casualty is fit to return to active duty.
Importantly, the military casualty can and often will experience multiple serious injuries [72, 111]. Thus, the ideal biomarker needs to assess specifically brain injury, and its diagnostic accuracy should be not affected by extracranial injury (i.e., polytrauma). Remarkably, a recent study on moderate-mild TBI (GCS ≥ 12) demonstrated that the levels of GFAP in serum were able to significantly differentiate not only between TBI patients and uninjured controls but also between TBI patients and trauma controls (trauma injury without evidence of acute brain injury) [112]. Similar results were demonstrated with serum levels of UCH-L1 differentiating between mTBI, moderate TBI, and uninjured and trauma controls [113].
Furthermore, due to the logistic constraints faced on a battle field, any diagnostic method must meet additional requirements sometimes not faced in civilian medical care and may require different platforms for different levels of care. A handheld system would be most suitable for use by emergency medical personnel or personnel deployed in the remote and more austere locations on the battlefield. These systems would ideally use to screen for the presence of TBI, while a small single assay point of care system could be used in a field hospital to screen for the presence of mild-moderate TBI and differentiate the level of severity for all TBIs.
Current research and development in the field of brain injury biomarkers give hope the development of an objective diagnostic test for the presence of TBI. Such test will significantly advance the management of civilian patients as well as assist in an accurate evaluation of TBIs experienced by military personal.
Having such a tool would assist military medics to objectively and quantifiably diagnose the presence and severity of TBI, provide advances in objective triage capabilities, and improve clinical management of in-theater TBI. Additionally, it would assist in making important decisions for the casualty’s treatment and ultimately affect the long-term health of the warfighters.
Diagnostic Markers of Sports Concussion
It is estimated that between 1.6 and 3.8 million concussions occur annually in the United States [134], and even this estimate may be low, because results of several studies suggest that sports concussions tend to be vastly under diagnosed and consequently poorly managed [96]. This has been a consequence of misconception that concussions are not serious injuries. However, in the past few years, mounting evidence indicates that structural and clinical sequelae are associated with concussions [98, 143]. Most commonly, post-concussive symptoms resolve within a short period (7–10 days), without permanent sequelae, but some athletes can have serious disabling long-term consequences including prolonged post-concussive symptoms, cognitive impairment, early onset dementia, movement disorders, psychiatric disorders, motor neuron disease, and chronic traumatic encephalopathy [51, 98]. In addition, a catastrophic injury, called the “second impact syndrome,” can occur when an athlete who has not recovered from an initial concussion sustains a subsequent blow to the head. The result of this trauma is either significant morbidity or death [162].
Therefore, this increasing awareness has spurred significant efforts to identify individuals with concussion in order to provide appropriate medical care and establish when an athlete can safely return to play after a concussion. Furthermore, it is important to identify individuals who are predisposed to sustaining a concussion or at risk for an adverse outcome after a concussion in order to prevent or at least minimize severe long-term consequences.
At the present time, the only diagnostic tests for brain injury available to physicians are expensive imaging modalities, such as computed tomography (CT) and magnetic resonance imaging (MRI). Unfortunately, these imaging modalities have suboptimal sensitivities for concussion (3–10 % for CT and 10–57 % for magnetic resonance imaging); studies are often not able to confirm mild TBIs and may only identify the very serious injuries that result in swelling and/or bleeding [8].
Therefore, multiple studies have been conducted to identify serum biomarkers that can offer superior diagnostic and prognostic information related to concussions. Furthermore, biomarkers may provide information regarding the molecular pathophysiology of concussive brain injury with practical implications for the management and treatment of athletes who suffered a concussion.
To date limited studies evaluating biomarkers in the setting of a concussion have been conducted, and most of these researches did not specifically evaluate patients with concussion but rather patients with mild traumatic brain injury.
Previous accurate research has shown that S100 has a negative predictive value of 90–100 % for predicting a normal head CT scan and therefore may be used to aid clinicians in emergency settings to determine when to order a CT scan in athletes with mild traumatic brain injury. Regarding the ability of S100B to predict persistent post-concussive symptoms, studies have provided conflicting results. Five studies have demonstrated that elevated levels of S100B are associated to increased incidence of persistent post-concussive symptoms in patients with a concussion [34, 146]. However, several studies were not able to find a significant association between elevated S100B levels and the development of post-concussive symptoms or persistent cognitive or functional abnormalities after a mild traumatic brain injury [8].
In addition, the lack of specificity of S100 for concussion is a major limitation. Elevated levels of S100B have been demonstrated in athletes without concussion who are participating in basketball, soccer, and hockey [140, 141]. Based on the available evidence, S100B does not have adequate specificity to assist with diagnosis of mild traumatic brain injuries in the setting of sports concussion, nor does it provide significant prognostic data related to post-concussive symptoms.
The sensitivity of NSE for mild traumatic brain injury is highly variable [46], and no correlation between post-concussive levels of NSE and persistent post-concussive symptoms was found [46, 139].
The ability of serum cleaved τ protein (CTP) to diagnose mild traumatic brain injury or predict prolonged post-concussive symptoms after concussion has been evaluated in four studies [9, 70]. No significant difference in serum levels of CTP between patients with mTBI and control was found [88]. CTP concentrations did not correlate with prolonged post-concussive symptoms [9, 88], and did not predict abnormal findings on head CT scans in patients with mTBI [70, 88].
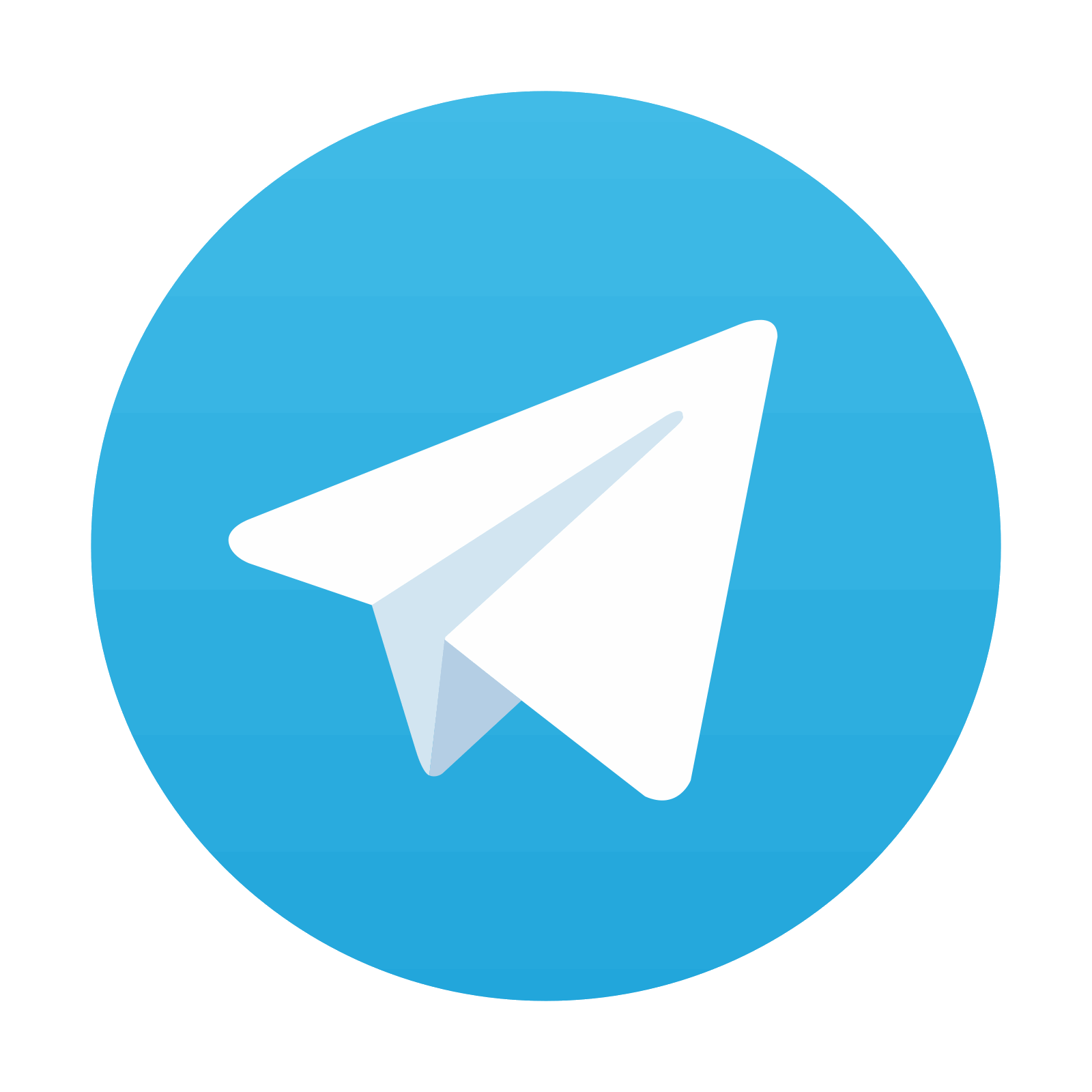
Stay updated, free articles. Join our Telegram channel
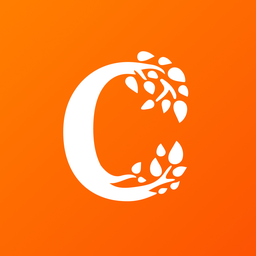
Full access? Get Clinical Tree
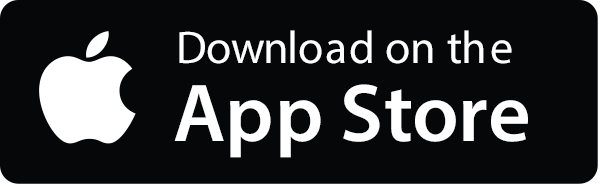
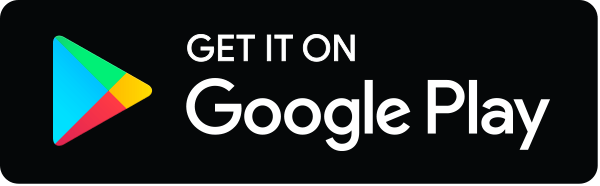