CHAPTER 10 Angiogenesis in Meningiomas
INTRODUCTION
To obtain nutrients, tumor cells initially rely on passive diffusion from their microbioenvironment.1 In 1947, Algire2 suggested that the formation of new blood vessels is required for progressive tumor growth. Folkman3 proved this theory in 1971 and demonstrated that solid tumors were absolutely dependent on angiogenesis for growth beyond a diameter of 2 mm. Angiogenesis research will probably change the face of medicine in the next decades, with more than 500 million people worldwide predicted to benefit from pro- or anti-angiogenesis treatments.4
Meningiomas are no exceptions to these fundamental rules of angiogenesis.5 Meningiomas derive their blood supply predominantly from meningeal vessels originating in the external carotid circulation. An additional supply from cerebral–pial vessels is present in approximately 60% of patients.6 Meningiomas are of variable vascularity, ranging from sparsely vascularized to highly vascular angiomatous meningiomas.7–9 They further display variable degrees of peritumoral brain edema, ranging from absent to life-threatening conditions.10 In contrast to gliomas, there is no scientifically proven association between edema and histologic grade in meningiomas.11 Nevertheless, extent of angiogenic activity during the meningioma formation and progression is certainly important in the surgical and endovascular management and recurrence of these tumors, regardless of their anatomic location.
BASICS OF VASCULOGENESIS AND ANGIOGENESIS IN NORMAL AND PATHOLOGIC CONDITIONS
The first vascular network in the embryo is established by the process of vasculogenesis at the time when somites begin to form. In the mouse the first blood islands arise by in situ differentiation from the extraembryonic mesoderm around E7.0 to 7.5. T’hey are located between the two germ layers and form the inner layer of the yolk sac.12–15 The extraembryonic yolk sac circulation, the primitive heart, and the primary vascular plexus inside the embryo, as the dorsal aortae, and the vitelline veins, thus form by the process of vasculogenesis. While this first vascular plexus is still emerging, early signs of its remodeling, especially inside the embryo, are visible. Network remodeling is defined as rearrangement of number or location of vascular segments to establish a functional adaptation without measurable network expansion.16–18 Vascular fusion reduces the number of segments and gives rise to larger vessels. At other places larger vessels disappear and/or are remodeled into a network of smaller ones subsequently increasing the number of segments.19,20 These processes lead to transformation of the uniform primary plexus into the secondary one that is more complexly structured. Further expansion of the primary and secondary vascular plexus occurs by the process of angiogenesis. Angiogenesis refers to blood vessel formation from preexisting ones.20 It implies two distinct mechanisms: endothelial sprouting and intussusceptive microvascular growth (IMG). Angiogenesis establishes the circulation in so far avascular regions. Concerning the formation of vascular networks in the forming organs, some are established by vasculogenesis, for example, lung and spleen, while others, such as the brain, are derived from angiogenesis.
Vasculogenesis
The first descriptions of the in situ formation of blood vessels in living animals, some of them derived from manipulations of the early chicken embryo and in vivo microscopic observations, date back to the 19th century.21,22 Sabin23 contributed the most detailed analysis of the process of vasculogenesis and clearly separated it by definition from angiogenesis.
Mesodermal cells in the extraembryonic yolk sac that are termed hemangioblasts give rise to the blood islands. These cells proliferate and differentiate to form the precursors of the endothelial cells of the vessel wall, the angioblasts, and the precursors of the hematopoietic cells located in the lumen. Fusion of the blood islands results in the primary vascular plexus.24,25 Inside the embryo, cells of the proximal lateral mesoderm assemble symmetrically at the lateral sides of the embryo to form the “preendocardial tubes”.24 The latter fuse at the anterior intestinal portal. The fused region forms the endocardium of the heart. Closely related to heart formation is the formation of the two ventral and dorsal aortae. These vessels develop by assembly of angioblasts to form four major channels. The two dorsal aortae fuse later in development to give rise to one single vessel. The vitelline or omphalomesenteric arteries arise from the distal parts of the dorsal aortae. They fuse with the yolk sac vessels similar to the two vitelline veins that form by splitting of the sinus venosus area of the developing heart. Mesodermal cells of the allantois give rise to the umbilical vessels. This demonstrates that formation of the large intraembryonic vessels is very early depending on the morphogenetic steps of vessel fusion and splitting that cause further growth and remodeling by the process of angiogenesis. Angioblasts can also migrate inside the embryo to form vascular plexus at distant locations. Chick-quail grafting experiments suggest that two types of intraembryonic hemangioblasts exist; one derived from the splanchnopleuric mesoderm can produce hematopoietic cells, unlike the other that originates from the somatopleuric mesoderm.26 Vascularization of organs derived form mesoderm and endoderm (as the lung and spleen) occurs primarily by vasculogenesis.25
Vasculogenesis is subsequently induced by the endoderm and mesoderm.25–28 Vascular endothelial growth factor (VEGF) is expressed in the extraembryonic visceral endoderm and in the extraembryonic mesoderm when the first blood islands emerge. At day 8.5, when the intraembryonic vascular plexus begins to form, surrounding endodermal cells exhibit strong VEGF expression, while the expression is moderate in mesodermal derived cells. Endothelial cells at this stage express the VEGF-A receptor flk-l (VEGFR-2, KDR) in a paracrine manner12,27 and flk-l upregulation is even induced by VEGF.29 Subsequently, gene targeting studies showed that flk-1−/− mice lacked vasculogenesis and failed to develop blood islands throughout the embryo and the yolk sac based on a lack of differentiation of mesodermal cells to form angioblasts. The embryos died between E8.5 and 9.5.30 The second tyrosine kinase receptor for VEGF, flt-t (VEGFR-1), is simultaneously expressed in endothelial cells during early embryonic development.31 Flt-1−/− mice also died by E8.0/E9.0 and lacked a proper organization of the blood islands.
lnactivation of a single VEGF allele in mice caused death of the embryos between El1 and El2. The VEGF+/− embryos exhibited a number of developmental anomalies, among them malformations of the heart, rudimentary dorsal aortae, and a reduced number of nucleated red blood cells in the yolk sac. This indicates that a threshold level of VEGF is required to maintain angioblast differentiation.32 Homozygous VEGF-A–deficient embryos that were completely derived from embryonic stem (ES) cells (generated by aggregation of homozygous VEGF-A–deficient ES cells with tetraploid embryos) died in mid-gestation (E9.5) based on even more severe cardiovascular defects. These results taken together indicate that the embryonic VEGF expression requires a precise regulation. In addition, other growth factors, such as transforming growth factor β (TGF-β), have also been linked to yolk sac hematopoiesis and vasculogenesis.12,16
Angiogenesis
Angiogenesis, the formation of blood vessels from preexisting ones, consists of two distinct processes: sprouting of endothelial cells and splitting of vessel lumens by IMG. Organs derived from the ectoderrn–mesoderm, such as the brain and neuroectoderm, are vascularized by angiogenesis.16
The sprouting mode of angiogenesis
One of the first descriptions of the sprouting process dates back to Galen of Pergamon (c. A.D. 130–200), who compared the developing embryo to a plant that grows along with the branching umbilical veins.33 More recently, a precise concept consistent with sprout formation has been elaborated using a variety of models. The most important ones are the chicken chorioallantoic membrane (CAM) and the corneal pocket.34,35 The sprouting process consists of several consecutive steps that have been described by Ausprunk and Folkman35 and many other authors:36,37
(Note that steps 1 and 11 are specific for tumoral, such as meningioma, conditions.)
The intussusceptive mode of angiogenesis
IMG has been detected in a variety of tissues and organs, in embryonic as well as adult angiogenesis.17,38,39 In addition, its existence has been proven by the demonstration of different mechanisms of its cellular implementation by in vivo video microscopy using the chicken CAM and tumor xenografts as model systems. These mechanisms were confirmed by the analysis of sequential serial sections using light- and electron microscopy.17,18
The following steps are common to all mechanisms of IMG analyzed so far:16–18,38,39
ln the adult situation, as in tissue repair or tumor angiogenesis, periendothelial cells of the vessel wall (fibroblast-like cells, pericytes, and smooth muscle cells) are involved in IMG.38,39 Fibroblast-like cells that expressed endothelial-specific markers such as platelet–endothelial cell adhesion molecule (PECAM), flt-l, and tie-2 were detected in tissue repair and tumors.
Vascular network remodeling
Remodeling is necessary to optimize the functional adaptation of the newly formed network.42,43 It implies addition of new vascular segments (vessels), but also the deletion of previous ones.16,17 Remodeling also includes the differential growth of segments, a process termed pruning.20 Pruning can occur by generation of new endothelial cells in the vessel wall. The size of segments is also increased by their fusion, and conversely the division of segments decreases their sizes. The number of segments is inversely affected by these two processes. Thus remodeling and pruning occur simultaneously.18
Vascular network remodeling also occurs as a response to divergent flow conditions. Numerous angiogenic molecules, for example, platelet-derived growth factor B (PDGF-B), and transcription factors are expressed by endothelial cells, whereas their receptors are up-regulated in perivascular cells in the vessel wall after the alteration of shear stress profiles.44 Endothelial cells in vitro that are exposed to large shear stress gradients respond with increased cell division and motility in the vicinity of flow separation.45 Network remodeling is also linked to maturation and stabilization of the vessel wall in larger vessels that is based on the recruitment and differentiation of mesenchymal cells or fibroblasts to form pericytes and smooth muscle cells.18,46
The molecular regulation of angiogenesis
In general, the molecular regulation of angiogenesis is the regulation of its distinct steps. Thus an angiogenic molecule promotes endothelial cell proliferation, migration, or tube formation while an inhibitor interferes with these steps. Concerning IMG, the ability to induce stretching and thinning of endothelial cells and movement of the endothelial layer is critical. Induction of cell membrane fusion to form transcellular holes in endothelial cells is another requisite as well as matrix proteolysis, collagen fibril synthesis, and recruitment of periendothelial cells. To date, a number of angiogenic growth factors have been identified. The most prominent ones are fibroblast growth factor 1 and 2 (FGF-1, acidic and FGF-2, basic), platelet-derived growth factor (PDGF), hepatocyte growth factor (HGF, scatter factor), VEGF-A, VEGF-B, VEGF-C, transforming growth factor α (TGF-) and interleukin 8 (IL-8).47 In addition, an important role in angiogenesis has been established for the tie/Angiopoietin and the Eph-B/ephrin-B system of tyrosine kinase receptors and their ligands.46,48,49
VEGF-A and its receptors (flk-l and flt-1)
Similar to its role in vasculogenesis, VEGF-A is also responsible for the regulation of angiogenesis. Many studies have demonstrated, mostly in vitro, that VEGF promotes migration and tube formation in endothelial cells. The VEGF family members have differing capabilities to promote these functions.50,51 VEGF+/− embryos die between day 11 and 12 from ubiquitous defects in their vasculature. These defects include abnormally large vessels in the yolk sac and inside the embryo accompanied by tissue necrosis, a lack of fusion of the vitelline veins with the yolk sac vessels, and failing growth of vessels from the perineural plexus into the neuroepithelium of the brain.32 This confirms the importance of VEGF for embryonic angiogenesis. Interestingly, high expression levels are detected in organs where vasculogenesis is the leading mechanism of vascularization, while lower levels are found in organs that receive their vascular supply by angiogenesis.12 Whereas the flk-1 receptor (VEGFR-2) is dominant during early vasculogenesis, flt-l (VEGFR-1) is prominent during remodeling of the primary vascular plexus and subsequent angiogenesis. Flt-l−/− mice thus exhibit large, dilated vessels in the yolk sac and throughout the embryo instead of a plexus of smaller ones.52
The Angiopoietin/tie system
The tie receptors form another family of receptor tyrosine kinases. They are important for the regulation of angiogenesis together with the ligands of the tie-2 receptor, the Angiopoietins. The tie tyrosine kinase receptors, like the VEGF receptors, form the only receptor families that are predominantly expressed on endothelial cells.53 Homozygous mutant mice deficient of the tie-l or tie-2 receptor undergo severe vascular malformations and die between days E13.5 and P0 (tie-1) and E9.5 and 10.5 (tie-2).54 This suggests that both receptors play a critical role in embryonic angiogenesis. The detailed analysis of tie-2 receptor deficient embryos revealed malformations of the heart derived from a lack of interaction between endocardium and myocardium. Abnormally large vessels were detected in the extraembryonic yolk sac circulation, and in many vascular networks throughout the embryo, for example, the perineural and intestinal vascular plexus.16,54 Ultrastructural analysis demonstrated a failure of endothelial cell stretching (interaction with their extracellular matrix) in numerous locations as well as a lack of recruitment of periendothelial cells (pericyte-, smooth muscle-, and myocardial cell precursors). This caused an aberrant morphology of tissue folds and tissue pillars that were not able to divide the embryonic vessels as they did in the control embryos with subsequent collapse of some vascular segments. In addition, the formation of vascular loops that formed in situ according to the principles of IMG was disturbed. Thus loop systems that invaded the neuroectoderm and the surrounding mesenchyme from the perineural plexus in the control embryo were diminished in number and rudimentary in structure in the tie-2 mutant.18 The only mechanism by which vessels could remodel in the tie-2 knockout embryo was based on a flat collapse of opposing vessel walls. This was frequently followed by thinning and cell membrane fusion of the opposing endothelial cells with formation of two transcellular holes. Invasion of these holes by matrix elements formed a new branching point and divided the vessel segment permanently into two parts. However, the collapsed area was much larger in diameter as compared to the region of insertion of tissue folds that divided vessel segments in the normal case. Thus the newly separated segments were much smaller and located more distant to each other as compared to wild-type segments. These malformations of the vascular network demonstrated that the embryo was very likely unable to perform a physiologic gas exchange. Necrotic cells detected throughout the embryonic tissues support this interpretation.18 Homozygous mice for a targeted mutation of Angiopoietin-1 (Ang-1), a ligand that specifically activates the tie-2 receptor, exhibited a corresponding phenotype.46 Angiopoietin-1 is expressed on periendothelial cells, suggesting a paracrine regulation.18 In addition, Ang-1 is chemotactic for endothelial cells, but does not cause endothelial cell proliferation or tube formation in vitro.55 These data taken together support the hypothesis that the Angiopoietin-1/tie-2 system promotes IMG rather than endothelial sprouting. Although Koblizek and colleagues56 detected endothelial sprouting induced by Ang-1 in a specialized in vitro system of angiogenesis, they confirmed the notion that Angiopoietin-1 was only weakly mitogenic for endothelial cells. Further, Ang-1 increases the survival of networks exposed to VEGF or acidic FGF and prevents apoptotic death triggered by growth factor withdrawal. In addition, transgenic overexpression of Ang-1 in the skin of adult mice results in larger, more numerous, and highly branched vessels as compared to controls.57 The view that Ang-1 cooperates with VEGF to ensure embryonic angiogenesis16,18,22 was confirmed in the cornea micropocket assay in the adult. In this system the combination of Ang-1 and VEGF resulted in an enhanced capillary density and an increased luminal diameter of the basal limbus artery as compared to the effect of VEGF alone.58
Angiopoietin-2 also binds specifically to the tie-2 receptor; however, it has no activating effect and consequently blocks Ang-1 activity. Thus mutant mice that overexpress the Ang-2 ligand exhibit a phenotype corresponding to the tie-2-or Ang-1–deficient mice.59 Interestingly in, situ hybridization during the ovarian an cycle in adult mice showed that Ang-2 is coexpressed with VEGF within a region of vessel growth. presumably promoting angiogenesis by endothelial sprouting. Ang-2 expressed in the absence of VEGF was related to an area of vessel regression.59 Corresponding data were obtained in the corneal micropocket assay where Ang-2 together with VEGF caused the formation of elongated vessels and “isolated sprouting cells located at the tip of developing capillaries.”58
The tie-1 receptor tyrosine kinase was originally associated with a survival function for endothelial cells and a stabilization effect on the vessel wall. Mutant mice deficient in the tie-1 receptor exhibited holes in vessel walls and endothelial cells that appeared to be necrotic.54 A more recent investigation of these mice demonstrated significantly increased numbers of blood vessels throughout all tissues and organs investigated. Ultrastructural analysis revealed that many endothelial cells were in a “hyperactive state” compared to others that matched those in the control animals. These hyperactive endothelial cells were extremely stretched with numerous cellular filopodia that projected into the vessel lumen and were even connected to the opposite vessel wall. These cells also showed frequent intra- and transcellular holes at places not related to tissue pillar separation. These data taken together support the hypothesis that the tie-1 receptor could be an inhibitor of angiogenesis that would inverse the functions of the tie-2/Ang-1 system. It likely also influences the process of cell membrane fusion that is necessary for all mechanisms of IMG.18 The recent analysis of double mutant embryos that lack both tie-1 and tie-2 receptors revealed a phenotype largely corresponding to the one of tie-2–deficient mice with an increased severity and an earlier onset of the mutant characteristics.
The ephrin-B/Eph-B system
Recently another receptor tyrosine kinase family, the Eph-B receptors and their ligands, the ephrins, have been shown to promote embryonic and tumorigenic angiogenesis.48,49 lnterestingly, the ephrins are not soluble ligands, but they are membrane attached and their binding to the receptor requires cell–cell contacts.48 A bidirectional signaling between ligand and receptor is suggested.60 The ephrin-B2 ligand was located strictly on arterial endothelial cells, while the corresponding Eph-B4 receptor marked only venous endothelial cells. Analysis of ephrin-B2 knockout mice showed vascular defects comparable to those of the Ang-1 or tie-2 receptor-deficient mouse, such as the persistence of the primary uniform vascular plexus.48 From these studies it appeared that arterial and venous sites are at least partly genetically determined before the typical physiologic characteristics have developed. While in the control yolk sac arterial and venous vessels were alternating the ephrin-B2–deficient mice showed a strict separation of arterial and venous sides. Similar to the situation in the Ang-1/tie-2 knockout mice, periendothelial cells were missing or they remained distant to the endothelial layer.The branches of the anterior cardinal vein were also affected and lacked signs of remodeling.48 In addition, in another investigation a more widespread expression of the Eph-B receptors has been suggested. The expression of Eph-B receptors and the ephrin-B2 ligand on periendothelial cells was demonstrated and a possible role of the Eph-B/ephrin-B system in remodeling of the primary vascular plexus confirmed.49 To investigate whether the function of endothelial ephrin-B2 can be compensated by ephrin-B2 that is expressed on perivascular cells, transgenic mice were generated in which ephrin-B2 is specifically deleted in the endothelium and endocardium of the developing vasculature and heart. The resulting phenotype corresponded to the one of the ephrin-B2 null mutants, indicating that periendothelial ephrin-B2 expression is not sufficient to compensate for the endothelial and endocardial ephrin-B2 loss.61
lnteractions of VEGFs, angiopoietins, and ephrins
Taken together these data show that VEGF might be one of the leading gowth factors involved in the regulation of vasculogenesis. The Angiopoietin/tie system is activated later and cooperates with VEGF during the period of angiogenesis. While VEGF promotes endothelial cell migration, proliferation, and tube formation, its effect on intussusception still is not known. Yet the flt-1−/−and the VEGF+/− phenotypes resemble those of the Ang-l /tie-2 knockout. Whether this could be derived from a disturbance of the cellular mechanisms of IMG needs further investigation. Ang-1 and its tie-2 receptor are clearly involved in the regulation of IMG, as has been established in two detailed studies.18,46 Concerning the role of Ang-2 and its cooperaion w ith VEGI, the interpretation of the present data has suggested that endothelial sprouting be promoted. This is based on the conclusion that Ang-2, because it interferes with the effect of Ang-1 and is coexpressed with VEGF in areas of vessel growth, must enhance the established function of VEGF, which is to promote endothelial sprouting.59 However, this implies that VEGF is not effective in the mechanisms of IMG. Further, because the vessels that developed during the ovarian cycle reflect the morphology of loops rather than of single blind-ending sprouts that are not perfused, it can be imagined that in situ loop formation by IMG could be a relevant mechanism. These questions will of course need careful investigation in the future by analysis of serial sections at the histologic and ultrastructural level.
Interestingly, the Ang/tie system and the ephrin-B/Eph-B system have similar roles in angiogenesis. The Angiopoietins might transport signals from mesenchymal cells to endothelial cells through a larger intercellular space to ensure their “communication and connection.” This facilitates varying mechanisms of IMG that require the involvement of periendothelial cells to guarantee the formation of stable tissue folds and ITSs for vascular network formation, growth, and remodeling. During IMG the endothelial layer actually retreats toward the mesenchyme to form tissue folds and loops. The ephrin-B/Eph-B system could exert a similar effect. In addition, it allows for “communication” between endothelial cells of opposite sides of the circulation, subsequently establishing direct cellular connections between the arterial and venous sides. These arterial and venous endothelial cells come into contact during IMG within the capillary areas of the circulation that lack supporting cells.39,62
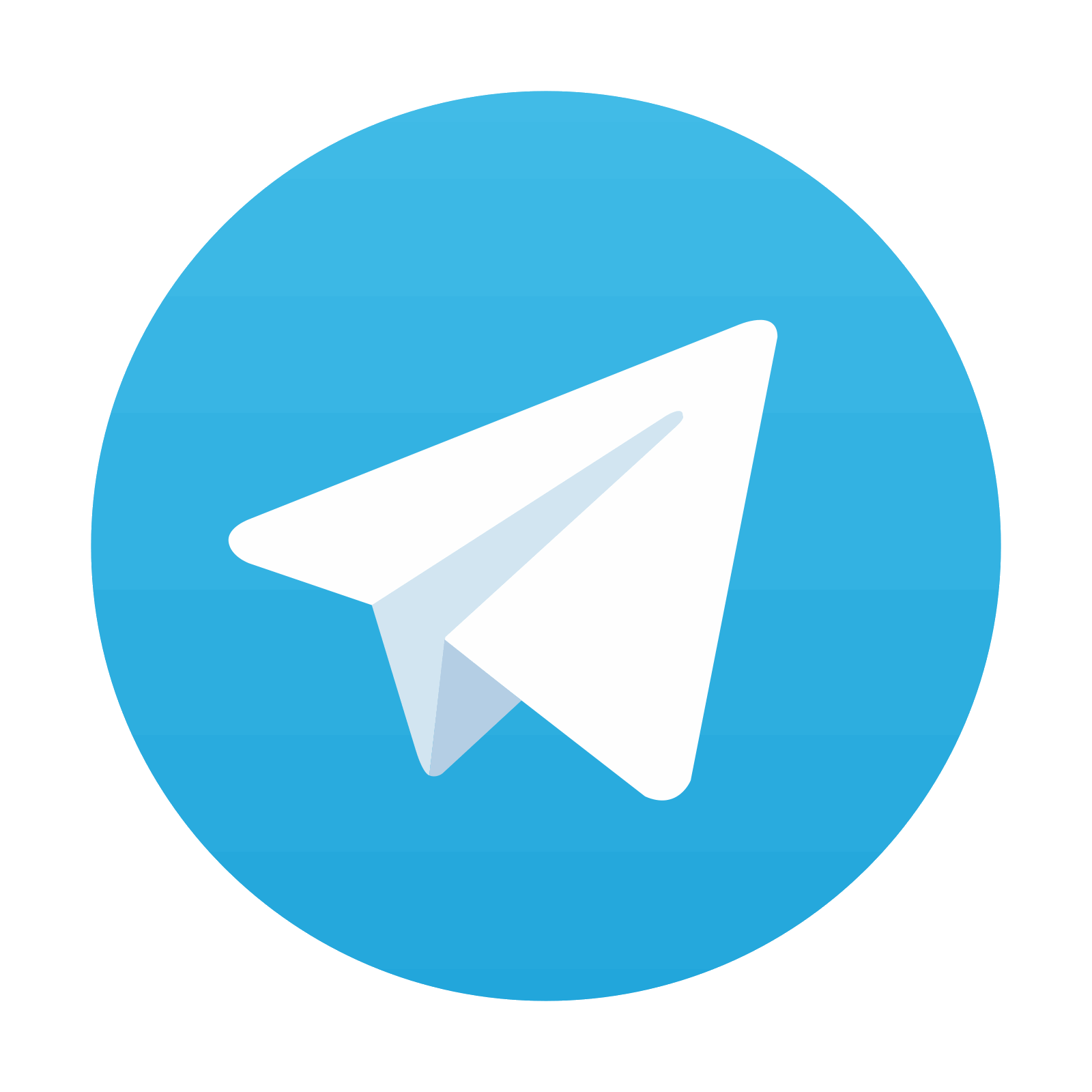
Stay updated, free articles. Join our Telegram channel
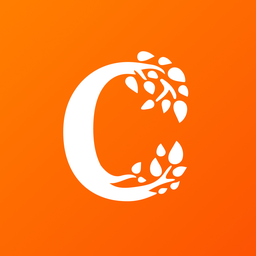
Full access? Get Clinical Tree
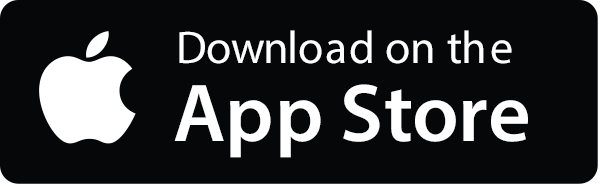
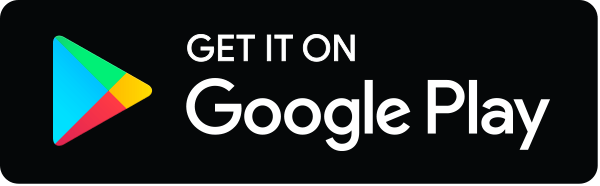