muscle strength reduction in the ankle plantiflexor muscle, while 12–16 weeks lead to a reduction of muscle strength over the same muscles. Therefore, early therapy is essential for the patient’s recovery. One of the most beneficial treatments involves physical therapy in conjunction with pharmacological treatment. A variety of treatment options are available and clinical experience has shown that a multimodal approach has many benefits [58]. Therefore, researchers in the BCI field are also directing this technology in clinical applications such as neurorehabilitation.
BCIs used in neurorehabilitation applications could be used to treat a wide variety of neurological or neuropsychological disorders. However, this chapter will focus only on BCIs that aid in the recovery of motor functions. Ideally, the main goal of such a BCI in neurorehabiliation would be to totally restore the patient’s motor function but this is not always possible. Yet a lot of BCI studies have been focused on developing applications for establishing communication channels, environmental control and control of devices that substitute motor function to improve life quality of people with disabilities [66].
The development of BCIs oriented to neurorehabilitation therapy should be focused on classifying the patients by the extent, rather than the etiology, of their disability [68]. This distributes potential BCI users into three reasonably distinct groups: (1) people who have no detectable remaining useful neuromuscular control and are thus totally locked-in; (2) people who retain only a very limited capacity for neuromuscular control, such as weak eye-movements or a slight muscle twitch; and (3) people who still retain substantial neuromuscular control and can readily use conventional muscle-based assistive communication technology.
In order to obtain successful results, it is important to know what occurs inside the brain after damage has occurred due to a traumatic brain injury. Experimental evidence has shown that the brain possesses cortical plasticity, which can be defined as the ability of the nervous system to respond to intrinsic or extrinsic stimuli by reorganizing its structure, function, and connections [29]. Several years of research have demonstrated that the neuroplasticitiy is stimulated by a learning process [24]. Moreover, this learning process can be encouraged in three different manners:
The user realizes the movement. The most common effect after a cerebrovascular damage is motor disability contralateral to the brain lesion. The suggested physical therapy is a technique applied to recover motor function, and it is focused on task specific training that involves the affected limb [99]. The realization of a real movement is the most logical manner to stimulate the neuroplasticity process. Therefore, this technique has been widely applied in the rehabilitation process [50]. During rehabilitation therapy, the patient should be assisted by a therapist who helps him/her to realize the desired movement. However, recently different technologies have been developed in order to assist the patient in this rehabilitation process [19, 34]. The rehabilitation that implies real movement is directed not only to patients who retain a substantial neuromuscular control, but also for patients with limited control.
The user imagines the movement. Motor imagery (MI) can be defined as a dynamic state during which a given action is mentally simulated by the patient. Considering the physiological bases, movement execution and motor imagery share common mechanisms. In both cases, event-related desynchronization of mu (or Rolandic) and beta rhythms over the contralateral side of the brain with respect to the movement are present. The region of the brain that is most activated during motor imagery is the prefrontal cortex, which is involved in the movement planning [32]. This kind of learning has demonstrated to activate the cerebellar and cerebral networks [56]. The patient could implement the imagination in two different manners: “first person perspective,” or motor-kinesthetic, and “third person,” or visuo-spatial perspective. For the purpose of obtaining results in neurorehabilitation, it is important that the patient carries out the first type of motor imagery because only motor-kinesthetic imagination modulates the corticomotor excitability [98]. This type of physical therapy is more effective for patients with severe motor disorders and impairments, when physical exercise is no longer possible.
The user observes the movement. The idea that observation of movement is an alternative technique for neurorehabilitation was firstly studied in monkeys. Excitability in a section of neurons was noticed when a monkey observed another individual (congener or the trainer) performing a movement [33, 93]. This behavior was also observed in healthy humans [38]. The results of this experiment demonstrate that excitability of the motor system increases when a subject observes an action performed by another individual. Furthermore, the pattern of muscle activation evoked by transcranial magnetic stimulation during action observation is very similar to the pattern of muscle contraction present during the execution of the same action. This activity is produced by mirror neurons which are a particular class of visuomotor neurons that fire when an individual visualizes another performing an action, and these have a particular role in action understanding. A more detailed study of mirror neurons in both monkeys and humans is presented in [94]. A study with patients revealed that recovery of motor functions after stroke is positively influenced by the observation of the action since such observation induces reactivation of the motor areas [37]. This type of physical therapy that encourages the learning process might be more useful to a patient who is locked-in or when a patient has spent some time without performing the movement and thus has problems to imagine the movement (as was described in the imagination of movement section, the kinesthetic part is very important). Also, this kind of learning can be combined with both, imaginary or real movement. In combination with imaginary movement, it is an additional approach to provide feedback to the patient. In combination with real movement, the patient can actually realize the movement and consequently this helps to obtain better results in the rehabilitation therapy.
Since the performance of a real movement was the most obvious answer to treat motor disorders, physical neurorehabilitation was the first technique that was studied and used in this field. The rehabilitation process was managed by a physiotherapist who made the patient perform several motor exercises to improve his/her condition [28]. With recent advances in technology, neurorehabilitation has become a multidisciplinary field. The medical field is its kernel but currently, neurorehabilitation can be supported by the use of devices and supplementary technologies in order to achieve a high level of performance and patient recovery. The use of the first rehabilitation devices, such as threadmills and other exercising machines [31], was followed by the appearance of more complex devices, such as exoskeletons. There are numerous researchers worldwide exploring this field of studies. In [34], a thorough review on lower limb rehabilitation is given among, in which several kinds of robotic lower limb devices and rehabilitation techniques are described. There are also a lot of studies focused on upper limb rehabilitation by robotic systems as exhibited in [19]. The neurorehabilitation improvement can also be achieved by other means, such as using different kinds of muscle stimulation (magnetic, electrical, and ultrasound) [100, 109] or using new emerging technologies.
With the current appearance of motor imagery and visual movement neurorehabilitation techniques, BCI technology has become applicable in the rehabilitation field. BCIs provide a means of recording electrical brain impulses related with motor imagery tasks. With a proper processing and classification algorithm, it is possible to detect several motor tasks from brain signals while a person is imagining the movement. In [90], the researchers could recognize between two mental tasks related with the imagination of left-hand movement and right-hand movement with an average classification accuracy of
with three users. With this level of classification, a BCI system could provide visual feedback about the imagined movement or even to control a device according to the desired movement. It has been demonstrated that visual feedback related with a desired movement combined with imaginary movement provides improved results in rehabilitation over using only imaginary movement [80].

By combining a BCI with other rehabilitation systems, it is possible to considerably improve rehabilitation strategies. In [49], a hierarchical BCI system is used to control a planar robot in a 2-dimensional plane by the classification of 2 mental tasks related with right- and left-hand imagery movements. This system simultaneously provides motor imagery and visual neurorehabilitation to a patient. There are more complex studies, such as the Smart Wearable Robots with Bioinspired Sensory-Motor Skills (BioMot Project) [13] with the goal of developing an exoskeleton that receives data from several physiological factors such as electroencephalographical (EEG) and electromyographical (EMG) signals. In this project, EEG data is used to detect the intention of the initiation or termination of a movement, to measure changes in velocity and direction/orientation, and to evaluate the attention level of the patient and the possible appearance of obstacles during the rehabilitation process. In this case, the use of an exoskeleton allows physical rehabilitation and the use of a BCI includes the patient’s desires and mental state in the rehabilitation process, which makes the patient take a more active part of his/her recovery. With these kind of systems, the same physical movements performed by patients and triggered by their desires are used as visual movement feedback. This combines the three types of neurorehabilitation strategies mentioned on this chapter.
2 Problems and Need on Neurorehabilitation
In the beginnings of rehabilitation, the obvious way to tackle a motor dysfunction was the first strategy mentioned in the last section, the performance of real movements guided by clinicians. For that reason, physiotherapists and other experts on the field have developed several works related with this strategy. In order to rehabilitate a patient, the method followed is not the only trouble to get over. Although it was well known that the origin of neurologic disabilities is placed at the central nervous system (CNS), conventional therapies used in neurorehabilitation two decades ago were mainly focused on providing sensory feedback [78] and performing real movements. Main problems found by clinicians on this matter are mentioned below.
Physical problems
Economical problems
Facilitating functional restitution. The principal mechanisms implicated in motor recovery involve enhanced activity of the primary motor cortex induced by active motor training [22]. A simultaneous activation of sensory feedback circuits and primary motor cortex may reinforce cortical connections by Hebbian plasticity principles and support functional recovery [78]. Assuming that the connection between peripheral muscles and the sensorimotor cortex has been disrupted in a CNS lesion, it is necessary to develop new clinical approaches that encourage patients on paying closer attention to the motor tasks performed [7]. This may constitute neurofeedback interventions with the capability of inducing neural plasticity [44]. An emerging intellectual paradigm for neurological recovery that includes neural regeneration, repair, and dynamic reorganization of functional neural systems, as well as increasing awareness of behavioral principles that may support best return to function and freedom, has brought forward treatments based on experience-dependent learning, neurophysiologic stimulation, and a combination of these concepts [9]. BCI systems employ neurofeedback to enable operant conditioning to allow the user to learn using it. Neurofeedback strategies ranging from sensory feedback to direct brain stimulation are also being used [97]. There is not conclusive data in the literature about the superiority of one type of feedback. Some study suggests better results in patients who used a haptic feedback versus visual or auditory feedback [85]. Several studies in last years have been developed to demonstrate the clinical efficacy of BCI in neurorehabilitation, and they have had significant results and positive correlations with functional outcome measures as Fugl-Meyer Assessment [4–6, 25, 91]. Since the performance of physical movements by neurological patients is often not possible, alternative strategies are needed. Motor imagery combined with a BCI system represent a new approach to access the motor system that can be used for rehabilitation at all stages of stroke recovery [7, 95] avoiding the dependance on residual motor performance.
Maladaptative changes. The neuroanatomical, neurochemical, and functional changes that occur during the plasticity reorganization facilitate the recovery of affected functions (adaptative plasticity) and may hinder the development of others (maladaptative plasticity). Usually it is difficult to avoid that patients develop compensatory movements in order to be functional, but these new skills are reflected in motor cortex like maladaptative changes, hindering the normal evolution of neural plasticity [72]. Several studies developed during BCI interventions have showed results in terms of clinical motor improvements correlated with neuroimaging techniques. [25] presented a case report of a BCI intervention using functional magnetic resonance (fMRI) showing evidence of recovery as a result of adaptative brain plasticity in the injured hemisphere of a stroke patient. [101] reported another fMRI trial in stroke patients compared with a robotic intervention, finding higher functional connectivity changes in the BCI control group. Also, [52] developed an intervention with transcranial direct current stimulation (tDCS), showing an increase in the activation of cerebral patterns in the affected hemisphere too.
Patient’s motivation. Plasticity of central nervous system conditions include the importance of motivation and attention [29]. Active participation of patients throughout the rehabilitation process is a prerequisite to facilitate recovery [87]. Still these processes are sometimes repetitive and last long periods of time, making difficult to maintain the patient’s interest on recovery. BCI could be used to induce and guide activity-dependent brain plasticity by paying close attention to a motor task that requires the activation or deactivation of specific brain signal. [20] revealed an improvement in concentration and participation after a BCI intervention motivated by the recovery of skill lost after a neurological lesion. Entertainment-orientated BCI applications through motor imagery have typically had a lower priority in neurorehabilitation field, but it could be another future application [81].
Generalization of learning. The development of new technologies to facilitate the performance of evaluation and intervention procedures has stimulated research on novel rehabilitation paradigms and more effective rehabilitation strategies. More realistic scenarios are necessary to support learning processes and their incorporation into daily living. In this sense, the VR-based rehabilitation has been validated in the treatment of acute and chronic stroke and has been proved to be more effective than existing methods [103]. For the generalization of learning with BCI interventions, some works [5, 6] have made a follow-up after BCI interventions, showing adherence to therapy, as well as conservation of several improvements and gains in motor outcomes.
Physical effort required by clinical personnel during manual therapy. There is no clear evidence that better outcomes can be achieved using technology-assisted intervention modalities compared to conventional therapies, but an advantage of robotic devices is their possibility to provide intensive therapies. Cerebral plasticity is an individualized process limited in time, and there is enough data to conclude that repetitive, high intensity, task-orientated training is efficacious [73]. Still, these conditions are not possible with conventional therapies. Higher intensity of practice is proved to be an important aspect of effective physical therapy, and additional therapy time of 17 h over 10 weeks is necessary to find significant positive effects at body function level [102]. The current challenge for BCI technology in neurorehabilitation is to enable the effective clinical use of BCIs with minimal effort to install and manage the devices used to register EEG signals to solve several problems yet derived from comfort, signal-acquisition hardware, and usability [96].
Development of accurate assessment tools for the evaluation of neurological deficits. The motor cortex recovery is one of the biggest enigmas in neuroscience. Motor recovery patterns are heterogeneous, and until the last decade, the only prognostic indicator used was the degree of motor deficit [72]. The severity of the initial deficit and the improvement in the first weeks are the strongest indicators for a favorable outcome. Neural plasticity is an individualized process limited in time, so therapy should be regularly adapted and stopped if the deficit remains stable [73]. Imaging techniques are used to confirm neuroplastic changes produced by rehabilitation, still these techniques have some limitations in their use, mainly because of the costs, contraindications, and poor accessibility. Also, patients with an undesired evolution time are excluded from rehabilitation programs [111], so it is necessary to design new treatment strategies and sensitive measures of outcome predictions that facilitate the clinical decision-making process. Characterization of brain signals from electroencephalogram is feasible, and these signals can be analyzed in real-time, which is useful for control and self-regulation of brain function [97]. Another application of BCI interventions could be the analysis of the evolution of motor patterns activation during recovery.
Costs of equipments are another problem to solve. New rehabilitation devices like exoskeletons have high marketing costs. Currently, each exoskeleton costs around $100,000, which is why they are only being sold to rehabilitation centers and hospitals. Several individuals suffering from motor disorders have costs that sometimes exceed one million dollar over their life time due to their condition. Added to these costs, the acquirement of proper rehabilitation devices is a really hard task. Looking at the exoskeleton market, the cheapest offer seen on current days is the HAL equipment from Cyberdyne which rent you this exoskeleton for $1000 a month [30]. Even with these prices the complete acquisition of these systems becomes difficult for an average individual. It is necessary to develop new low-cost technologies. In a period of limited resources and continuous increase of health care expenditures, clinicians need to carefully evaluate the economic impact of their decisions. In the last decade, economists have been particularly productive in offering to the decision makers a set of tools able to compare costs and benefits of each single-medical procedure. Long-term therapies can also be considered a great effective learning environment for health professionals. Prolonged therapies can be considered an environment for experimenting “creative” solutions and approaches [17]. The characterization and management of electrophysiological signals from the central nervous system with EEG equipment have been reinforced by various characteristics that make the EEG optimal clinical evaluation tool. They are noninvasive, portable devices, and affordable. In this regard, different authors have used to assess the functional cortical reorganization after motor cortex lesion. The analysis revealed EEG characteristics and uses of different patterns of brain activation related to movement and neural mechanisms related to motor control. Furthermore, it has revealed that the activity-dependent plasticity occurs in the central nervous system throughout life and that, therefore, the study of their evolution can be a useful way to determine the functional consequences of neurological accident and its progression [72].
Logistic problems
Another drawback of new systems like exoskeletons is the limitation in their use. There is a large percentage of paraplegics who will not be able to benefit from this technology based upon their height, weight, and type of injury. As an example, ReWalk Robotics define that the individual using their exoskeleton ReWalk [110] must be between 157–193 cm in height, weight less than 100 kg, and must have strong upper body strength to use the device. Quadriplegics are individuals who cannot move their upper and lower body. Because of this, they will be a large category of people who cannot use this technology due to their physical limitations. Implementation of care activities into daily practice is essential to improve quality of care, but it is also challenging. There are people who need rehabilitation that due to the region they live or their physical limitations cannot move easily to the rehabilitation center. There is a branch of telemedicine called telerehabilitation focused on solving this problem by the use of the telecommunication technologies [42], but telerehabilitation based on the performance of real movements is still nearly impossible because the patient should have all the expensive equipments at home and the therapist will not be there if the patient health is in risk during some exercise.
3 Review of BCI Systems
A BCI, also referred to as a brain machine interface (BMI), gives a person the ability to communicate with and control a computer through his or her brain signals rather than using the peripheral nerves and muscles [105]. This technology is directed towards providing people with severely restricted mobility or suffering from motor neuron degenerative diseases the possibility of communicating through a software application or controlling a device without requiring continuous assistance. BCIs are also starting to be used by healthy individuals, in particular in gaming applications, and may be expected to become an alternative means of communication and control in the near future.
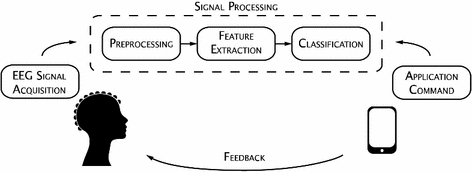
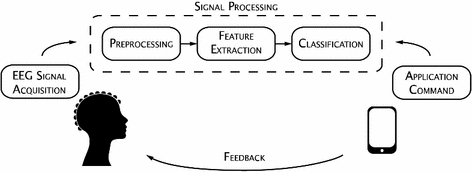
Fig. 1
General BCI process
3.1 A General Process of a BCI System
Noninvasive BCIs use electroencephalography to acquire the electrophysiological signals produced by the neurons and synapses of the central nervous system, and translates them into commands or messages to external devices. A typical process of a BCI system is shown in Fig. 1. This consists essentially of the EEG signal-acquisition stage, the signal processing stage, and the application interface.
The signal-acquisition stage involves the recording of brain activity using EEG equipment while the user performs a predefined task. Electrical brain activity is recorded as voltage fluctuations resulting from ionic current flows within the neurons of the brain by electrodes placed on the scalp [82]. Each electrode reflects the summation of the synchronous activity of a large number of neurons in that particular area of the brain. The 10–20 international electrode placement system [69] is a standard method used for electrode placement. It is desirable to choose only the most relevant and necessary EEG channels while recording data. Minimizing the number of electrodes makes the BCI system more portable, practical, easier, and faster to set up. Moreover, reducing the number of EEG signals results in a possible smaller feature space and less computationally complex signal processing, which in turn reduces the classification time and hence improves the information transfer rate of the BCI system. Brain signals measured on the surface of the scalp have amplitudes in the order of hundreds of microvolts and thus must first be amplified. Preprocessing is carried out to improve the quality of the raw EEG data and filter unwanted noise and artifacts. The data is then passed through a feature extraction algorithm that obtains relevant and discriminative information from the EEG data that characterizes the distinctive neurophysiological phenomena. These features are fed into a classifier that predicts the brain activity of the user. This predicted class is translated in real time into a particular application command providing direct feedback to the user.
3.2 EEG Control Signals Used in BCI Systems
There are various brain signals evoking distinctive characteristics that have been adopted as control signals for BCIs. These include:
Sensorimotor Rhythms (SMRs). These are oscillatory patterns of cortical activation and inhibition that can be observed over the sensorimotor cortex during an imagination or actual execution of a movement [79]. Movement-related activation of the sensorimotor cortex is associated with two oscillatory patterns which are event-related desynchronization (ERD) and event-related synchronization (ERS). An ERD is an amplitude decrease of rhythmic activity occurring during motor preparation and execution, and is dominant in the alpha frequency band (around 8–12 Hz), while an ERS is an amplitude increase present after movement and is found in the beta frequency band (around 16–22 Hz). SMRs have the advantage of being well localized on the sensorimotor cortex; i.e., movement of a particular body part can be observed in the corresponding area of the cortex. This spatial information is used in motor imagery-based BCIs to distinguish between different motor imagery tasks [63, 88, 106]. The fact that motor imagery requires no actual muscular activity to modulate brain patterns is a great advantage for assistive BCIs. Nevertheless, substantial amount of training to voluntarily modulate SMRs and gain control of a motor imagery-based BCI is required.
Slow Cortical Potentials (SCPs). These are slow-voltage shifts of the mean potential measured on the cortex. A negative shift of the cortical potentials correlates with increased neuronal activity, while a positive shift correlates with a decreased activity [26]. SCPs can be self-regulated through a thought-translation device (TTD) [15], which involves a training process that rewards the trainee for achieving the desired brain potential change [105]. SCP self-regulation training may take several months and depends on numerous factors such as the trainers psychological and physical state. The long-training period necessary for accurate control of SCP-based BCIs is their main disadvantage. BCIs based on SCPs also have a relatively low-information transfer rate since typically several seconds of recording are required to discriminate between a positive and negative SCP.
Visual Evoked Potentials (VEPs). These are electrical potentials recorded over the visual cortex and evoked by a visual stimulation. In [92], phase-locked occipital responses were studied by presenting a stimulus light modulated at a certain frequency. The EEG waves evoked by these stimuli produced a stable VEP response proportional to the stimulus frequency, thus were termed steady-state visual evoked potentials (SSVEPs). SSVEP-based BCIs consist of flickering stimuli that induce SSVEPs at different frequencies, each associated with a particular command of an application. The user focuses his/her attention on the target stimulus while the BCI system analyzes the ongoing EEG, extracts relevant SSVEP features to predict the target stimulus, and executes the command linked to that particular stimulus. Some of the advantages of SSVEP-based BCIs are that few electrodes over the occipital region are required since SSVEP is well localized; SSVEPs are less susceptible to EOG and EMG artifacts, and the user does not require training to evoke an SSVEP response. The disadvantage of SSVEP-based BCIs is that they require eye-gaze shifting.
P300 Potential. This is a significant mid-central peak observed approximately 300ms after an infrequent stimulus is evoked among a series of routine stimuli. The Farwell–Donchin (oddball) paradigm [41] was developed to evoke P300 potentials during which subjects are presented with a random sequence of two types of stimuli: the target stimulus (or oddball) appearing rarely in the sequence and the nontarget stimulus appearing more often in the sequence. P300-based BCIs are limited to a low-information transfer rate [81]. In addition, the P300 potentials are not well localized on a specific region of the cortex [26] and thus require a larger number of electrodes than that necessary for a BCI system based on SSVEPs. The advantage of a P300-based BCI is that users do not need to train to control the EEG signals. However, offline training sessions are generally still required to train the BCI algorithm that detects P300 potentials which are known to vary between users [26].
3.3 Applications of BCI Systems
The BCI research is currently focused at developing clinical applications to serve as direct control to assistive technologies and as a form of rehabilitation [68]. BCI communication systems are vital applications for patients with strong disabilities. Various spellers using different EEG control signals have been developed [1, 27, 83, 104] although they are still limited by relatively low-information transfer rates. Restoration of movement control with BCI systems have also been studied by evaluating navigation systems through virtual environments [40, 63], control of mobile robots [71], and motorized wheelchairs [43, 62, 74]. BCI systems have also demonstrated potential use for controlling the movement of electrically driven prosthesis and orthosis [48, 75, 89]. The implementation of the discussed BCI applications would be personalized for locked-in patients or patients with severe motor impairments based on the patient’s alertness, attention, visual and auditory capabilities, and higher cortical functions [68]. Novel hybrid BCI systems have been developed, which are either a combination of different types of BCIs [2, 89], or a combination of control channels including a BCI channel and any other biosignal, such as electromyographic (EMG) or other assistive technology input device [70]. The innovation of portable and relatively cheap EEG systems [61], although not reliable for critical applications as a medical-grade EEG system, have triggered great interest for healthy users, such as BCI computer gaming applications [55, 57, 84]. Furthermore, BCI technology is envisioned in the near future to be further developed in other fields such as robot control performing in dangerous or extreme environments, vehicles, as well as attention monitoring of aircraft pilots and drivers. Despite the fast-growing technology, many limitations such as the accuracy, speed, practicality, reliability, and cost of current BCIs must be overcome before these can be used outside the laboratory.
4 BCI and VR as a Tool for Neurorehabilitation
BCI technology was focused primarily on developing assistive devices for people with limited mobility. However, recent progress in this field shows the potential use of novel applications in neurorehabilitation. Thus, BCIs could also be beneficial for users with disorders such as stroke, autism, and attention deficit hyperactivity (ADHD) [14]. Such BCIs ensure the participation of the patient during rehabilitation to stimulate brain plasticity, thereby significantly enhance rehabilitation therapy. Similarly, VR could increase the participation of the patient during rehabilitation, thus strengthening the benefits of the treatment. Typical physical rehabilitation therapy is intensified with VR by providing the patient with useful stimuli related to the function that is being rehabilitated. VR also has the advantage of modifying the environment which the patient interacts with during rehabilitation, thus introducing motivating situations that promote the participation of the patient [51]. Considering the benefits of these two technologies, merging them in a BCI-VR system could enhance todays neurorehabilitation. This section provides an overview of recent studies related to BCI-VR systems, in particular for applications in neurorehabilitation. The benefits and limitations in current systems will also be discussed.
4.1 Current BCI-VR Systems
Lately, research groups have combined BCI technology and VR to create various BCI-VR prototype systems. These studies have demonstrated that VR is a useful tool for analyzing and developing BCIs, since it allows performing safe and controlled experiments. The interactions between BCIs and VR can be broken down into basic tasks [59, 66], such as navigating the position of a camera [67], selecting or manipulating virtual objects [3], and controlling an application [35]. The first task can be controlled typically with one to three commands by means of different BCI paradigms, such as SSVEP [39] and motor imagery (MI) [3, 67], while the other tasks are based mostly on the P300 and SSVEP paradigms [35]. The selection of paradigms affects the intuitive and natural control of the BCI, while aiming to avoid fatigue to the user. A recent review [66] presented different BCI-VR application studies. This review discussed the impact of VR and its future applications in the BCI field context. However, there is lack of information on current BCI-VR systems developed for neurorehabilitation applications. Hence, state of the art in BCI-VR systems for neurorehabilitation will be discussed in the following subsection. An overview on current BCI-VR studies is first given.
One of the most important application for people with motor impairments that BCI-VR research has been focused on is mobility. Presently, the wheelchair is the most important technology that provides individuals who suffer from limited motor function such as spinal cord injury (SCI) mobility [16] because of the simplicity of its movement patterns. It has already been demonstrated in [62] that a tetraplegic can use a self-paced BCI to control a wheelchair in a virtual street using feet MI. A wheelchair displacement was produced in proportion to the duration of MI detected from the user’s EEG signals. The subject’s task was to reach the end of the street and stop at each one of the fifteen avatars found for 1 s and encouraged to communicate with them. In two days, the subject was able to stop at 90 % of the avatars, achieving in some runs a performance of 100 %. This BCI-VR application is appealing for wheelchair-bound people, as they can try a prototype system in a safe environment before it is actually developed. Eventually the goal is to control an actual wheelchair outside the lab in real circumstances. In [23] a similar system was further developed to increase the degrees of freedom in both direction and speed of the wheelchair to provide a more realistic mobility application for the user. Currently, efforts are being made in implementing augmented reality in a telerehabilitation environment for the training of wheelchair use [21].
Other studies have focused on mainly on the design of the VR using different BCI paradigms. For example, in [39] an SSVEP-based BCI system was developed in which the flickering stimuli that evoked SSVEPs were embedded in a virtual environment. This study proposed 3D SSVEP stimuli within the 3D graphics of VR or augmented reality (AR) [39]. In a first study with seven healthy subjects, the application was one among three VR scenarios, while in a second study with three healthy people the application was an AR scenario. The first VR scenario showed an avatar on a first-person perspective that had to push correctly one of two buttons that had at their side-squared stimuli that flickered at different frequencies. During this task, the user had to focus on the stimulus associated with pushing the correct button and to achieve as many correct activations as possible in a fixed time. The other VR scenarios had an avatar which the user had to navigate through a slalom or an apartment scenario. Three stimuli where placed at the left, top, and right of the scene associated with turning to the left, walking straight, and turning to the right avatar control commands. The slalom had a fourth stimulus for stopping the motion. The goal of these tasks was to follow a predetermined path in a fixed time. The average positive predictive value (PPV) for the VR scenarios was above
, while for the AR scenario, two of the three subjects could complete the task on time. These results suggest that this 3D virtual environment could elicit SSVEP, and therefore further BCI-VR realistic applications could be developed.

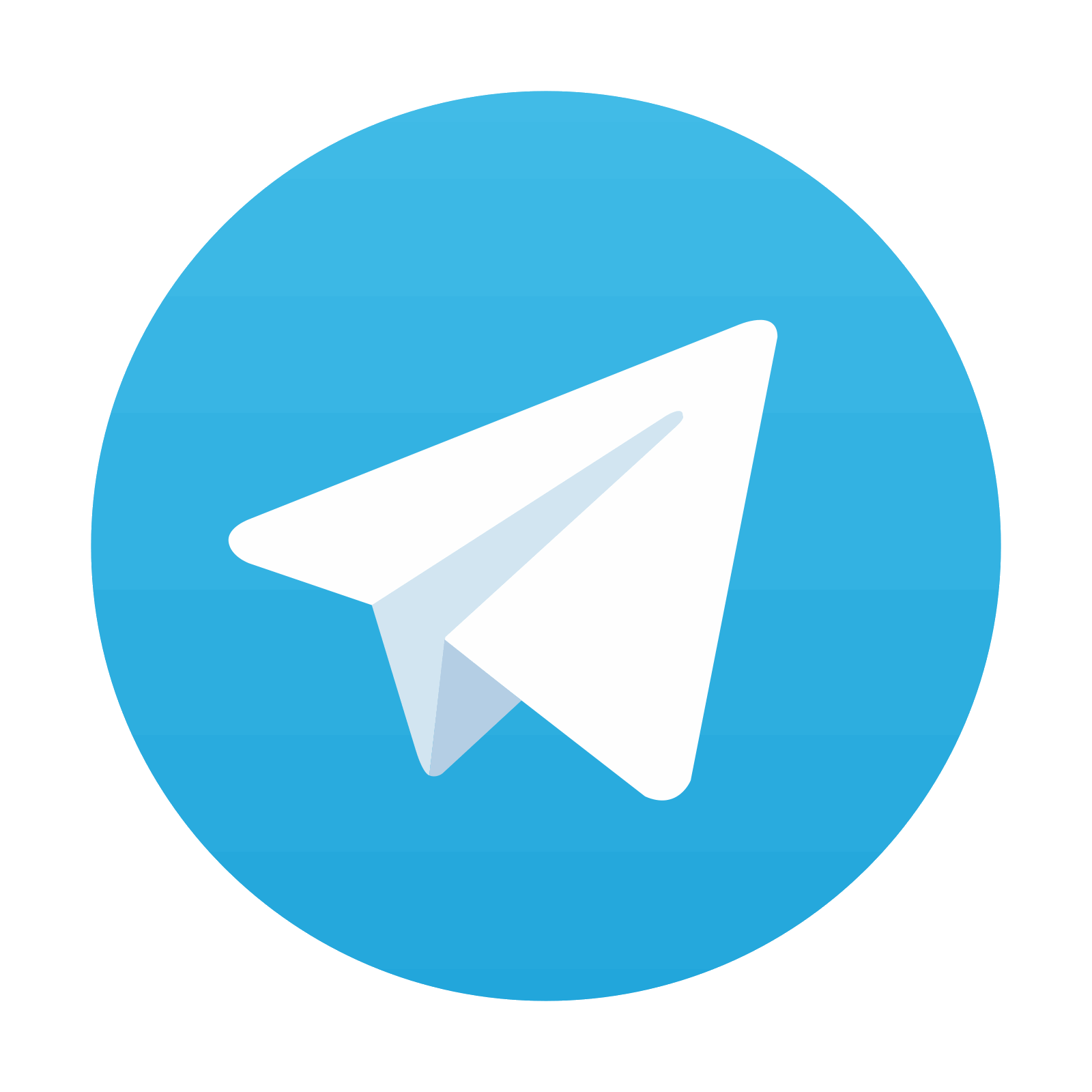
Stay updated, free articles. Join our Telegram channel
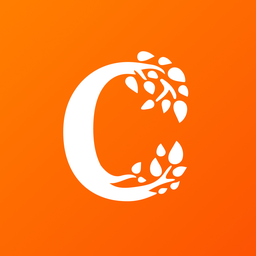
Full access? Get Clinical Tree
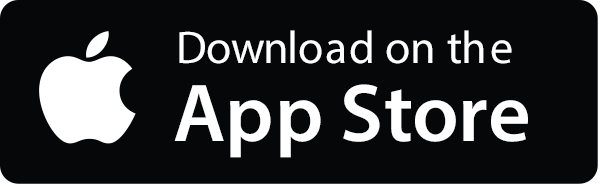
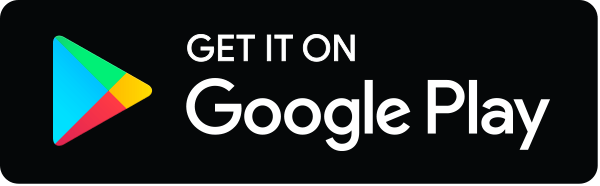