Abstract:
This chapter presents a systems model of the postural control system and explains how to use the model as a framework to understand postural control system impairments and their consequences. These negative effects include deficient sensorimotor balance skills, which in turn lead to functional activity limitations and participation restriction. An extensive set of objective balance measures is described with guidance for appropriate test selection and interpretation. Using a task-oriented approach to intervention, this chapter explains how to plan and progress balance exercise programs that target sensory inputs and organization, automatic postural responses (APRs) and anticipatory postural adjustments (APAs), and voluntary balance control within salient, real-life functional tasks. The clinician is provided with a comprehensive and flexible view of intervention emphasizing the need to treat impairments, teach sensorimotor balance skills, and train functional abilities.
Keywords:
activity limitation, anticipatory postural adjustments, automatic postural responses, balance, base of support, body structure and function impairments, center of gravity, impairment, limit of stability, motor learning stages, participation restriction, sensorimotor skills, sensory conflict, sensory environment, strategies, systems model or systems approach, volitional postural movements
Objectives
After reading this chapter the student or therapist will be able to:
- 1.
Describe both central and peripheral sensory and motor components of the postural control system.
- 2.
List common postural control system impairments and sensorimotor skill deficits found in patients with neurological problems.
- 3.
List commonly used balance tests, and distinguish which are appropriate for patients at low, moderate, and high levels of function.
- 4.
Differentiate how test results are used to identify postural control system impairments, sensorimotor skill deficits, and activity limitations that limit participation.
- 5.
Analyze the interaction of individual, task, and environmental factors that affect balance.
- 6.
Describe how to plan and progress balance exercise programs to increase the use of or compensation with available sensory inputs.
- 7.
Describe how to plan and progress balance exercise programs to facilitate anticipatory postural adjustments to prevent balance loss and provoke automatic postural responses to regain balance after unexpected disturbances.
- 8.
Describe how to plan and progress balance exercise programs to increase the control of center of gravity in upright postures and during gait.
- 9.
Describe how to increase the difficulty level of balance exercise programs in order to promote the automaticity of postural control during functional activities.
No matter what the neurological diagnosis, a disease or injury that affects the nervous system is likely to compromise one or more of the postural control mechanisms. For example, patients with such diverse diagnoses as stroke, head trauma, spinal cord injury (SCI), peripheral neuropathy, multiple sclerosis (MS), Parkinson disease (PD), cerebellar dysfunction, cerebral palsy, and Guillain-Barré syndrome all experience postural control problems. One common thread among these different medical diagnoses is the presence of postural control system impairments and, consequently, sensorimotor skill deficits, including imbalance and abnormal gait. Patients with different medical diagnoses may have the same postural control system impairments, and patients with the same medical diagnosis may have different postural control system impairments, depending on which portions of the postural control system are involved. To optimally understand and manage balance problems, assessment of each postural control system component and the interactive nature of the components is important. The traditional medical “diagnostic” model does not provide this information and is not the most beneficial model for planning balance rehabilitation interventions. The medical diagnosis is relevant: knowing whether deficits are permanent or temporary, and whether recovery or progressive decline is expected is critical. This medical prognostic information will assist physical and occupational therapists in goal setting and intervention planning.
The International Classification of Functioning, Disability and Health (ICF) model described in Chapter 1 and illustrated in Fig. 20.1 describes the interactions of body function and structure problems (impairments) and activity limitations as seen in patients with neurological disease or injury, and how these functional activity limitations restrict an individual’s ability to participate in life situations, thus decreasing quality of life. As valuable and beneficial as this model has been to rehabilitation practice, researchers and clinicians alike have had difficulty reaching a consensus about whether “imbalance” should be categorized as an impairment of body structure/function, or as an activity limitation. This is perhaps because the ICF categorizes some aspects of balance as impairments of body structure/function (e.g., “Involuntary movement reaction functions” including functions of postural reactions, righting reactions, body adjustment reactions, balance reactions, supporting reactions, defensive reactions) and other aspects of balance as activity limitations (e.g., “Mobility: Changing and maintaining body position,” including sitting, maintaining a sitting position, standing, maintaining a standing position, shifting the body’s center of gravity [COG]). To align with the ICF model, in previous editions of this chapter, I have used the term “balance impairments” despite concerns that neither category offered an optimal fit. The ICF model was not developed or designed specifically for use by movement system professionals such as physical therapists (PTs), occupational therapists (OTs), or adaptive physical education teachers with expertise in normal and abnormal motor control, motor learning, and motor development. I believe it is useful to adapt the ICF model for our purposes by adding a “sensorimotor skills” category in between body “structure/function impairments” and (more functional) “activity limitations” (see Fig. 20.1 ). Sensorimotor skills (i.e., perceptual-motor skills) are learned, goal-directed movement abilities that require the interaction and integration of sensory inputs, perceptual processes, motor planning, and movement execution. When we consider how or how well a patient moves and are concerned with the quality of their movement or movement patterns not only their outcome, we are engaged in sensorimotor skill analysis. Balance and gait fit very well into this category.

Within this adapted ICF model, body structure/function impairments (e.g., sensory loss, leg weakness, slow reaction time) lead to sensorimotor skill deficits (e.g., imbalance, hemiplegic gait patterns, abnormal synergies in reaching), which in turn lead to real-life, functional activity limitations (e.g., requires minimum assistance for wheelchair transfers, moderate assistance for limited household ambulation with a straight cane), culminating in participation restrictions and decreased quality of life. Application of this adapted ICF model to patient-centered rehabilitation care includes the following assessment sequence: (1) identification of the patient’s participation goals, (2) hypothesis generation about and evaluation of the functional activity limitations leading to participation restriction, (3) hypothesis generation about and evaluation of the sensorimotor skills leading to functional activity limitations, and (4) hypothesis generation about and evaluation of the body structure/function impairments leading to sensorimotor skill deficits. For example, a patient may wish to participate in a tour of historical buildings but cannot perform stair-climbing without either two handrails or assistance (activity limitation). The therapist hypothesizes that the patient’s dynamic balance is deficient and finds she cannot perform single-leg stance skills on either leg; further evaluation includes hypotheses and testing to reveal that she has weak hip extensors and abductors, and limited ankle dorsiflexion range of motion (ROM) bilaterally. Application of this adapted ICF model to the design of customized, individualized intervention programs thus requires that we treat impairments , teach sensorimotor skills , and train functional activity abilities if we wish to increase participation and improve quality of life. We cannot assume that doing one will automatically lead to another and should incorporate all three. For the patient described above, intervention would include treatment of impairments (e.g., closed-chain resistance exercises and ankle stretching), teaching dynamic balance skills (e.g., hands-free alternate toe touching on increasingly higher step stools, progressing to step ups/downs with dual-tasking), and training stair climbing (e.g., progressing to single or no handrail and counter-traffic).
Balance skill deficits negatively affect function, often reducing the individual’s ability to participate fully in life. These skill deficits often limit functional activity levels, produce abnormal compensatory motor behavior, and may require devices for support or assistance from another person. Falls can result when imbalance is severe, leading to secondary injuries. To avoid these consequences and advance the functional status of patients, therapists should understand both the demands that various environments and functional tasks place on postural control systems and the individual’s impairments and skill deficits that may diminish the ability of those systems to respond adequately.
Balance
Definitions of balance
Balance is a complex process involving the reception and integration of sensory inputs and the planning and execution of movement to achieve a goal requiring upright posture. It is the ability to control the COG over the base of support in a given sensory environment. , The COG is an imaginary point in space, calculated biomechanically from measured forces and moments, where the sum total of all the forces equals zero. In a person standing quietly the COG is located just forward of the spine at approximately the S2 level. With movement of the body and its segments, the location of the COG in space constantly changes. The base of support is the body surface that experiences pressure as a result of body weight and gravity; in standing it is the feet, and in sitting it includes the thighs and buttocks. The size of the base of support will affect the difficulty level of the balancing task. A broad base of support makes the task easier; a narrow base makes it more challenging. The COG can travel farther while still remaining over the base if the base is large. The “shape” of the base of support will alter the distance that the COG can move in certain directions.
Any given base of support places a limit on the distance a body can move without either falling (as the COG exceeds the base of support) or establishing a new base of support by reaching or stepping (to relocate the base of support under the COG). This perimeter is frequently referred to as the limit of stability or stability limit. , It is the farthest distance in any direction a person can lean (away from midline) without altering the original base of support by stepping, reaching, or falling.
Environmental context
This biomechanical task of keeping the COG over the base of support is always accomplished within an environmental context, which is detected by the sensory systems. The sensory environment is the set of conditions that exist, or are perceived to exist, in the external world that may affect balance. Peripheral sensory receptors gather information about the environment, body position, and motion in relation to the environment, and body segment positions and motions in relation to the self. Central sensory structures process this information to perceive body orientation, position, and motion and to determine the opportunities and limitations present in the environment. This central sensory integration process also estimates where we will be in the immediate future, permitting APAs. Gravity is one environmental condition that must be dealt with to remain stable. It is a constant condition for everyone except astronauts in space. Surface and visual conditions, however, may vary significantly and may be stable or unstable. Unstable surface conditions might include the subway, a sandy beach, a gravel driveway, or an icy parking lot. Common unstable visual conditions are experienced on mass transit, in crowds, or on a boat. Rapid head movements may render even a stable visual environment unusable for postural cues, and darkness may preclude the use of vision. The more stable the environment, the lower the demand on the individual for balance control. Unstable (extrinsic) environments place greater demands on the (intrinsic) postural control systems.
Balance is also affected by an individual’s intentions to achieve certain goals and the purposeful tasks that are undertaken. Volitional balance disturbances are self-initiated almost constantly, such as shifting from foot to foot, reaching for the telephone, or catching an object that is falling from a high shelf. Even reactions to involuntary balance disturbances, such as a slip or trip, are modified on the basis of the immediate task. A man carrying a bag of groceries who slips may drop the bag to reach with both hands and catch himself. If he is instead carrying his infant, he may reach with only one hand or even take the fall if by doing so he can protect the infant from harm. Often in real life we perform several tasks at once, such as carrying a laundry basket while walking or talking on a cellular phone while climbing a flight of stairs. When tasks are undertaken concurrently (dual-tasking or multi-tasking), attention must be divided between them, which may also affect balance abilities. This is especially true for individuals for whom balance skills are not automatic.
All of these variables—the location of the COG, the base of support, the limit of stability, the surface conditions, the visual environment, the intentions and task choices—are inconstant and produce changing demands on the systems that control balance. The integrity and interaction of postural control mechanisms allow a wide range of movement skills and real-life functions to be achieved without loss of balance.
Human control of balance
Early studies of postural control mechanisms using selectively lesioned cats and primates focused on reflexive and reactive equilibrium responses that are relatively “hard-wired.” These valuable studies brought to light certain stereotypical motor responses to specific sensory stimuli, such as the crossed extension reflex or tonic neck reflexes. There is no doubt that these reflexive and reactive responses—for example, the vestibuloocular reflex (VOR) and protective extension reactions—are foundational to normal postural control. However, the postural control system encompasses much more than these subcortically driven components. Balance abilities are heavily influenced by higher-level neural circuitry and other systems (e.g., cognitive, musculoskeletal) as well. In addition, the nervous system is influenced by and responsive to the demands placed on it by the tasks being accomplished and the environments in which those tasks are performed. All of these facets are included in a systems approach to dynamic equilibrium. Examination and intervention methods based on this systems model have consequently evolved. ,
The systems approach
The systems model for dynamic equilibrium recognizes that balance is a result of interactions among the individual, the task(s) the individual is performing, and the environment in which the task(s) must be performed. These interactions are represented in Fig. 20.2 . Within the individual, both sensory inputs and processing systems (left side of figure) and motor planning and execution systems (right side of figure) are critical. Both peripheral components (lower part of figure) and central components (upper part of figure) of the systems are involved in the cycle. The cycle is driven both by purposeful choices of the individual (tasks) and by demands placed on the individual by the environment. Successful function of the sensory systems allows recognition and estimation of body position and motion in relation to self and the world. The desired outcome from the motor systems is the generation of movement sufficient to maintain balance and perform the chosen, goal-directed task(s).

Peripheral sensory reception
The three primary peripheral sensory inputs contributing to postural control are the bilateral receptors of the somatosensory, visual, and vestibular systems. , Somatosensory receptors located in the joints, ligaments, muscles, and skin provide information about muscle length, stretch, tension, and contraction; pain, temperature, and pressure; and joint position. The feet, ankles, knees, hips, back, neck, and eye muscles all furnish useful information for balance maintenance. Somatosensation is the dominant sense for upright postural control and is responsible for triggering automatic postural responses (APRs) in almost all cases except a primary perturbation of the head. Somatosensory loss negatively affects balance. Loss of peripheral somatosensation occurs in patients with loss or disease of or injury to the peripheral sensory receptors or afferent sensory nerves. Examples include patients with diabetic neuropathy, peripheral vascular disease, spinal cord injury, MS, or amputation.
Visual receptors in the eyes support two functions. Central (foveal) vision allows environmental orientation, contributing to the perception of verticality and object motion, as well as identification of the hazards and opportunities presented by the environment. For example, a kayaker may see rocks in a stream as a hazard to be avoided, whereas a hiker who wants to cross the stream may see the same rocks as a welcome opportunity. Peripheral (ambient) vision detects the motion of the self in relation to the environment, including head movements and postural sway. Peripheral vision is largely subconscious, whereas central visual inputs tend to receive more conscious recognition. Both are normally used for postural control. Vision is critical for feedforward, or anticipatory, postural control in changing environments. This includes planning for functional movements such as reaching and grasping, and especially for successful navigation during gait. Vision loss also negatively affects balance. Loss of peripheral visual inputs occurs in patients with disease of or injury to the eyes or afferent cranial nerves. Examples include patients with cataracts, macular degeneration, glaucoma, or diabetic retinopathy.
The vestibular system provides the central nervous system (CNS) with information about the position and motion of the head. The position of the head in relation to gravity is detected through the otolith system. Horizontal and vertical accelerations, as in riding in a car or an elevator, are also detected by the otoliths. Movements of the head are detected through the semicircular canals. Head movement stimulates both sets of semicircular canals, so that the vestibular nerve on one side becomes inhibited while the other becomes excited. The vestibular system provides sensory redundancy in the information obtained from each separate vestibular apparatus. If the peripheral vestibular system is damaged on one side, the information can be captured by the intact canals on the opposite side. The vestibular system is critical for balance because it uniquely identifies self-motion as different from motion in the environment. Vestibular loss also negatively affects balance. Loss of peripheral vestibular inputs occurs in patients with disease of or injury to the peripheral sensory receptors or afferent cranial nerves. Examples include patients with head injury involving temporal bone damage, acoustic neuroma, benign positional vertigo (BPV), or Meniere disease. For a comprehensive review of the vestibular system and vestibular disorders, see Chapter 21 .
Orientation to the wider environment, primarily from vision, allows feedforward, or anticipatory, postural adjustments. Prior experience and high attentional capacity improve APAs significantly. Detection of head movement by the vestibular and cervical somatosensory systems and of body sway by somatosensory and peripheral visual systems provides feedback for APRs. Note that the better anticipatory abilities become, the fewer balance errors occur. Fewer balance errors means fewer losses of balance and a reduced need to produce rapid APRs.
Disease of or damage to any of the peripheral sensory receptors or afferent pathways impairs or removes the detection capabilities of the system rendering sensory information unavailable for use in postural control. Many patients with neurological diagnoses have peripheral sensory impairments.
Central sensory perception
The brain processes the environmentally available sensory information gathered by the peripheral receptors in varying degrees. This processing is usually referred to as multisensory integration or sensory organization. , Central sensory structures function first to compare available inputs between two sides and among three sensory systems. The somatosensory system alone is unable to distinguish surface tilts from body tilts. Also the visual system by itself cannot discriminate movement of the environment from movement of the body. The vestibular system by itself cannot tell if head movement through space is produced by neck motion or trunk and hip motion. Therefore the brain needs information from all three senses to correctly distinguish self-motion from motion in the environment.
How are sensory inputs from separate senses combined to form perceptions of position and motion? To explain this, consider the example of the movement of turning your head quickly to one side to look over your shoulder. When the head turns to one side, firing will increase in one vestibular organ and decrease proportionately in the other. This is known as push-pull function, and the information from each side is considered to “match” reciprocally. With the same example, if the eyes are open while the head moves, the rate of the visual flow will be equal and the direction of the visual flow will be opposite to the rate and direction of information from the vestibular inputs. The muscles on one side of the neck will shorten and on the other side will stretch. The inputs from these three systems are congruent. If both sides and all three systems provide compatible inputs, the process of sensory organization is simplified.
When changes in the environment occur, the relative availability, accuracy, and usefulness of information from the three sensory systems may also change. Sensory organization also includes an adaptive process, called multisensory reweighting, that permits the CNS to prioritize the sources of sensory information when environmental conditions change. , Available, accurate, and useful information is “upweighted,” whereas unavailable, inaccurate, or less-useful information is “downweighted.” For example, in dark environments vision would be downweighted and somatosensory and vestibular information would be upweighted. This adaptive process is imperfect, however, and balance is not as well controlled when any sense must be downweighted as it is when all three senses are available and accurate. Individuals with peripheral sensory loss or central sensory processing deficits may have difficulty reweighting quickly and fully. This impairs their ability to adapt to, and remain stable in, changing environments.
Sensory conflict can arise when information between sides or between systems is not synchronous. Sensory organization processing then becomes more complex because the brain must then recognize any discrepancies and select the correct inputs on which to base motor responses. The vestibular system may be used as an internal reference to determine accuracy of the other two senses when they conflict. For example, a driver stopped at a red light suddenly hits the brake when an adjacent vehicle begins to roll. Movement of the other car detected by the peripheral visual system is momentarily misperceived as self-motion. In this situation, the vestibular and somatosensory systems do not detect motion, but the forward visual flow is interpreted as backward motion. Because the brain failed to suppress the (mismatched) visual inputs, the braking response was generated. Note that “perception drives action,” thus perceptual errors lead to movement errors.
When the brain recognizes that the information coming from one sensory input is inaccurate or unavailable, as is the case when the perception of somatosensory information is diminished post-stroke, it must depend more on the remaining senses (in this case, vision and vestibular system) to determine position and motion in space. The brain then compares and uses information from senses it considers accurate for balance. An individual with the problem just described may compensate for the loss of somatosensory function by becoming visually dependent for balance during movement. If vision subsequently also becomes disrupted as this patient ages, his or her ability to orient in space will be further compromised. This will negatively affect balance and increase risk of falls.
Activities or environments that create sensory conflict or demand sensory resolution become more difficult to manage when the vestibular system is deficient or underused. These situations, such as going down stairs, riding escalators or elevators, walking on uneven ground, and making quick turns, are often avoided by patients with vestibular deficits. When sensory conflicts cannot be resolved rapidly, dizziness or motion sickness occurs.
Intrinsic central sensory processing impairments also can produce sensory conflict. An adult hemiplegic patient with pusher syndrome illustrates an inability to integrate visual, vestibular, and somatosensory inputs for midline and verticality orientation. Within a single system, discrepancies between the sides are also problematic. Unequal firing from opposite sides of the vestibular system, as in unilateral vestibular hypofunction, produces a mismatch that is subsequently interpreted as head rotation when head movement does not occur. This spinning sensation is known as vertigo. Vertigo is resolved if the brain is able to adapt to the mismatch. For further information on vertigo, refer to Chapter 21 .
Finally, the central processing mechanisms combine any available and accurate inputs to answer the questions, “What is my position and how am I moving now?” and “What will my position and motion be in the next moment?” This includes both an internal relation of the body segments to one another (e.g., head in relation to trunk, trunk in relation to feet) and an external relation of the body to the outside world (e.g., feet in relation to surface, arm in relation to handrail). CNS disease or trauma involving the parietal lobe may impair these processing mechanisms so that even available, accurate sensory inputs are not recognized or incorporated into determinations and estimations of current and future position and movement. , Impairments of central sensory processing may occur after stroke, head trauma, tumors, or aneurysms; with disease processes such as MS and Alzheimer disease; and with aging.
Central motor planning and control
Whereas sensory processing allows the interaction of the individual and the environment, motor planning underlies the interaction of the individual and the task. Aside from reflexive activity such as breathing and blinking, most motor actions are voluntary and occur because some goal is to be achieved. That is not to say that reflexes occur separately from volitional movements; for example, the VOR is active concurrently with visual tracking activity, but most actions occur because of some purposeful intent. These task intentions precede motor actions. , Wrist and hand movements vary depending on what is to be grasped (a cup vs. a doorknob); foot placement and trunk position vary depending on what is to be lifted (a heavy suitcase vs. a laundry basket). The initiation of volitional motor actions depends on intention, attention, and motivation. ,
Once a task goal (Where do I want to be? What do I want to do?) has been chosen, the next step in motor planning is to determine how to best accomplish the goal given the many options that are potentially available. For example, when the task demands fine skills or accuracy, the dominant hand is preferred; when the task involves lifting a large or heavy object, both hands are preferred. In addition to which limbs, joints, and muscles will be used, motor planning also adjusts the timing, sequencing, and force modulation of muscle activity. This can be demonstrated in various reaching tasks. Reaching to remove a hot item from the oven will occur slowly, whereas reaching to put an arm through a sleeve will occur more quickly. Optimal motor plans are developed with knowledge of self (abilities and limitations), knowledge of task (characteristics of successful performance), and knowledge of the environment (risks and opportunities).
The motor plan is generated in the cortex and refined in the basal ganglia, which responds to goal achievement with reward signals that increase motivation and the likelihood that the correct movement will be reproduced. Next, the motor plan must be transmitted to the peripheral motor system to be enacted. A copy of the intended movement plan is sent to the cerebellum during the transmission. When the movement begins, incoming sensory inputs (feedback) about the actual movements and performance outcome are compared with the intended movements and performance outcome. Movement errors (the difference between the intended and the actual movement) and performance errors (desired goal not achieved) are detected by the cerebellum and basal ganglia and transmitted to the cortex. Corrected motor plans are then formed in the cortex and transmitted for the next attempt to achieve the goal. This process of error detection and error correction is the foundation of motor learning.
Patients with CNS disorders often have central motor planning and control system problems. After a stroke, patients may have hypertonus and poor reciprocal inhibition; patients with head trauma may have difficulty initiating or ceasing movements; patients with PD exhibit bradykinesia; and those with cerebellar ataxia display force modulation problems.
Peripheral motor execution
Movement is accomplished through the bilateral joints and muscles. Normal joint ROM, muscle strength and power, and muscle endurance of the feet, ankles, knees, hips, back, neck, and eyes must be present for the execution of the full range of movements needed for normal balance skills. Decreased ankle dorsiflexion ROM, for example, restricts the forward limits of stability. Strength deficits are a primary cause of movement abnormalities in both CNS and peripheral nervous system (PNS) disorders. In addition, weakness may be the result of force modulation deficits or disuse. Balance is directly negatively affected by loss of muscle strength, power, and endurance. For example, weakness of the hip extensors and abductors will impede successful use of a hip strategy for upright trunk control. Low muscle power impedes the rapid generation of sufficient force to execute a successful APR. Initially adequate toe clearance may diminish with fatigue. Many patients with neurological issues also have stiffness and contractures as a result of persistent weakness or hypertonus. Restrictions in ROM, particularly extension, also limit balance skills.
The ability to achieve static postural alignment, although necessary for normal balance, is not sufficient to allow volitional functions. Adequate strength (to control body weight and any additional loads) through normal postural sway ranges is needed to permit dynamic balance activities such as reaching, leaning, and lifting. Postural control demands are increased during gait because the forces of momentum and the interaction between recruitment, timing, and velocity also must be regulated. Traditionally considered orthopedic problems, deficits in muscle strength and power, ROM, posture, and endurance have a negative impact on balance skills. Attention must be given to these musculoskeletal system problems in examination of and intervention for patients with neurological diagnoses.
Influence of other systems
Balance skills are also influenced by other systems . Attention, cognition and judgment, and memory are critical for optimal balance skills and are often impaired in hemiplegic and head-injured patients, as well as those who have progressive neurological disorders such as MS and Alzheimer disease. Attentional deficits reduce awareness of environmental hazards and opportunities, interfering with anticipatory postural control. When balance is threatened, an inability to allocate attention to the necessary task of balance versus a secondary, less necessary task increases the risk of falls. Cognitive problems such as distractibility, poor judgment, and slowed processing also increase the risk of falls. Memory loss may preclude recall of safety measures. Depression, emotional lability, agitation, or denial of impairments also can increase the risks for loss of balance. Fear of falling may lead to self-imposed participation restrictions that precipitate a downward spiral of more sedentary behaviors (leading to increased stiffness and weakness) and social isolation (leading to depression); all of these negatively affect balance and raise the risk of falls. In addition to having a direct impact on balance skills themselves, these cognitive and behavioral problems impede motor learning processes, which are crucial for the relearning of balance skills.
Constant cyclic nature
The systems model of postural control previously presented illustrates the constant cycle that simultaneously occurs at many levels. Attention and intention allow feedforward processing for active sensory search of the environment and motor planning, both of which are needed for anticipatory postural control. Movements are initiated and executed with resultant sensory experiences and error detection, or feedback. Successful movements are repeated and refined; unsuccessful ones are modified. The nature of this cycle presents the clinician with opportunities for intervention after the appropriate examination of sensory, motor, and cognitive functions. Through feedback and practice, balance skills can improve.
Motor components of balance
Reflexes
Many levels of neuromuscular control must be functioning to produce normal postural movements. At the most basic level, reflexes and righting reactions support postural orientation. The VOR and the vestibulospinal reflex (VSR) contribute to orientation of the eyes, head, and body to self and environment.
When motion of the head is identified by the semicircular canals, it triggers a response within the oculomotor system called the vestibulo-ocular reflex (VOR). This causes the eyes to move in the opposite direction of the head but at the same speed. Stimulation of the otoliths drives the eyes to respond to linear head movement. Quick movements of the head will trigger the VOR.
The VOR allows the coordination of eye and head movements. When the eyes are fixed on an object while the head is moving, the VOR supports gaze stabilization. Visuo-ocular responses often work concurrently with the VOR. They permit “smooth pursuit” when the head is fixed while the eyes move and visual tracking when both the eyes and the head move simultaneously.
The VSR helps control movement and stabilize the body. Both the semicircular canals and the otoliths activate and modulate muscles of the neck, trunk, and extremities after head movement to maintain balance. The VSR permits stability of the body when the head moves and is important for the coordination of the trunk over the extremities in upright postures. Righting reactions support the orientation of the head in relation to the trunk and the head position relative to gravity and include labyrinthine head righting, optical head righting, and body-on-head righting.
Automatic postural responses
At the next level, APRs operate to keep the COG over the base of support. They are a set of functionally organized, long-loop responses that act to keep the body in a state of equilibrium. , Functionally organized means that the responses, although stereotypical, are matched to the perturbing stimulus in direction and amplitude. If the stimulus is a push to the right, the response is a shift to the left, toward midline. The larger the stimulus, the greater the response. APRs always occur in response to an unexpected stimulus and are typically triggered by somatosensory inputs. Because they occur rapidly, in less than 250 ms, they are not under immediate volitional control.
Four stereotypical APRs have been described. Ankle strategy describes postural sway control from the ankles and feet. The head and hips travel in the same direction at the same time (“in-phase”), with the body moving as a unit over the feet ( Fig. 20.3 A ). Muscle contractile patterns are from distal to proximal (i.e., gastrocnemius, hamstrings, paraspinals). This strategy is used when sway is small, slow, and near midline. It occurs when the surface is broad and stable enough to allow pressure against it to produce forces that can counteract sway to stabilize the body. Ankle strategy is typically used to control anterior-posterior sway, because most of the degrees of freedom at the ankle are in this direction.

Hip strategy involves postural sway control from the pelvis and trunk. The head and hips travel in opposite directions (“out-of-phase”), with body segment movements counteracting one another (see Fig. 20.3 B ). Muscle contractile patterns are from proximal to distal (i.e., abdominals, quadriceps, tibialis anterior). This strategy is observed when sway is large, fast, and nearing the limit of stability or if the surface is too narrow or unstable to permit effective counterpressure of the feet against the surface. Hip strategy is used to control both anterior-posterior and medial-lateral sway. Hip strategy in the medial-lateral direction involves weight shifts from foot to foot; any patient with difficulty weight-shifting quickly and accurately will have difficulty with medial-lateral hip strategy.
Suspensory strategy involves a lowering of the COG toward the base of support by bilateral lower-extremity flexion, or a slight squatting motion (see Fig. 20.3 C ). By shortening the distance between the COG and the base of support, the task of controlling the COG is made easier. This strategy is often used when a combination of stability and mobility is required, as in windsurfing.
Stepping and reaching strategies involve steps with the feet or reaches with the arms in an attempt to reestablish a new base of support with the active limb(s) when the COG has exceeded the original base of support (see Fig. 20.3 D ). A successful stepping strategy (sufficiently large and fast) is the best way to avoid a fall after a slip or trip.
Misconceptions about these APR strategies are common. First, these strategies do not function in daily life as separately as they are described in the early research literature. In quiet standing, for example, frequency analysis of unperturbed postural sway in healthy adults reveals that both ankle and hip strategies occur in combination, simultaneously. In perturbation studies, mixed use of strategies is often seen unless the perturbation is clearly below or above certain-sized thresholds. Second, these strategies occur in response to disturbances from all directions, not just in pure anterior-posterior or medial-lateral directions. Third, although these strategies are stereotypical in humans, great individual variation in strategy selection and performance comes from other influential factors. For example, many people use stepping strategy for most perturbations unless specifically instructed not to step or unless the conditions do not permit a step. An anxious person may reach or step much sooner than a relaxed person with similar physical deficits. Last, all these strategies do not occur in sequence with every balance disturbance. , In other words, individuals normally do not try ankle strategy and wait until it fails before trying hip strategy, then wait until it fails before trying stepping strategy (although early learning in children may involve such exploration). Because these responses must occur extremely rapidly to prevent balance loss, such a sequential approach would be inefficient and ineffective. Instead, the normal adult response is the near-immediate emergence of the single strategy best suited to the particular perturbation, the limitations of the individual, and the conditions in the environment.
Abnormal use of APRs is often observed in individuals with neurological disorders. Patients with vestibular deficits typically rely on ankle strategy, which permits the head to remain aligned with the body and sustains congruence between vestibular and somatosensory inputs. Use of hip strategy may be modified or limited because when the head is moving in the opposite direction as the COG, vestibular and somatosensory inputs are not congruent. Activities that require use of hip strategy, such as standing in tandem or on one leg, can be a problem for patients with bilateral vestibular loss or an uncompensated vestibular lesion. However, some cases involve excessive use of hip strategy on a level surface (when an ankle strategy would suffice). This may reflect abnormal integration of the somatosensory and vestibular information. If peripheral somatosensation is impaired, as in diabetic neuropathy, or central sensory weighting of somatosensory inputs is inadequate, hip strategy may dominate.
Patients with somatosensory loss, distal lower extremity weakness or hypertonus, restricted ankle ROM, and/or reduced limits of stability typically rely on hip strategy. This occurs because the patient cannot feel the surface or the feet well enough to modulate foot pressure against the surface, because the person cannot rapidly generate sufficient force against the surface with the ankle muscles, or because restricted ankle ROM prevents COG sway. The use of hip strategy is normal when the COG is at or near the limits of stability and a step is either not possible or not desired.
When the brain perceives that hip or ankle strategy will not be efficient enough to control the movement of the center of pressure, or if conditions and instructions permit a stepping response, stepping strategy may be preferred. Individuals who are fearful of falling often perceive even slight body sway as threatening instability. They may use stepping and reaching strategies exclusively whether or not these “rescue” strategies are actually necessary.
Anticipatory postural adjustments
APAs activate muscles in a manner similar to APRs, but they occur before the actual disturbance. If a balance disturbance is predicted, the body will respond in advance by developing a “postural set” to counteract the coming forces. For example, if an individual lifts an empty suitcase thinking it is full and heavy, the anticipatory forces generated before the lift (to counter the anticipated weight) will cause excessive movement and brief instability. Failure to produce properly calibrated anticipatory adjustments increases the risk of sudden balance loss, creating the need to use rapid, reactive APRs to prevent a fall. For patients with deficits in reaction time or APRs, superior use of anticipatory postural control can help the patient avoid the unexpected perturbations that make APRs necessary.
In balance laboratories, APAs are studied using electromyography so that muscle activity before observable movement can be measured. In the clinic, problems with anticipatory adjustments may be observed when the patient fails to counteract a predicted disturbance, such as “don’t let me push you backward,” or fails to integrate postural control tasks during other activities; this can include the inability to step smoothly over an anticipated obstacle during gait or inability to maintain sitting balance when both arms are intentionally lifted overhead.
Patients with neurological disease or injury involving the cortex may have difficulty recognizing the need for and/or producing APAs. For example, individuals post-stroke or post–traumatic brain injury (TBI), or with MS, PD, or Alzheimer disease. Age-related white-matter changes in fall-prone older adults may also contribute to deficient APAs. Note that the motor learning needed to know when to generate and how to calibrate APAs requires practice and experience. Patients who are learning a novel task like a wheelchair “wheelie”—even patients without cortical involvement (e.g., individuals with SCI or Guillain-Barre)—need extensive repetition to be able to develop successful APAs for that task.
Volitional postural movements
Volitional postural movements are under conscious control. Weight shifts to allow an individual to reach the telephone or put the dishes in the dishwasher, for example, are self-initiated disturbances of the COG to accomplish a goal. Volitional postural movements can range from simple weight shifts to complex balance skills of skaters and gymnasts. They can occur after an external stimulus or be self-initiated. Volitional postural movements can occur quickly or slowly, depending on the goal at hand. The more complex or unfamiliar the task, the slower the response time. Use of a variety of movements that might successfully achieve a goal is possible. Volitional postural movements are strongly modified by prior experience and instruction. Automatic and anticipatory postural responses allow the continuous unconscious control of balance, whereas volitional postural movements permit conscious activity. This level of postural motor control is the most frequently tested and treated in clinical practice, but it is by no means sufficient by itself to produce normal balance.
Clinical assessment of balance
Objectives of testing
The patient interview should include determination of participation restrictions and the patient’s goals. Then, when present, activity limitations need to be identified and measured. Functional scales (e.g., Functional Independence Measure) are typically used to determine the presence and severity of these limitations, not necessarily why those limitations exist. From these functional tests, decisions can be made about whether intervention is required and, if so, what tasks need to be practiced. If intervention is indicated, clinicians must make judgments about what should be included in the intervention plan. Further testing to identify and measure sensorimotor skills deficits and body structure/function impairments is then necessary to know what systems are involved. A comprehensive evaluation of balance includes functional activity abilities/limitations, sensorimotor skills, and postural control system impairment tests.
No single quick-and-easy test of balance can adequately cover the many multidimensional aspects of balance, although many such tests have great value as screening tools. However, a comprehensive test battery, called the Balance Evaluation Systems Test (BESTest), based on the systems model has been developed that provides clinicians with a thorough examination covering the six major components of the postural control system ( Fig. 20.4 ). The BESTest takes more time to administer than, for example, a single-leg stance test, but results from the BESTest give the clinician a far more complete and accurate picture of the patient’s balance deficits than any single-item test or screening test can. Armed with these results, the clinician can develop interventions specifically targeted to the deficient systems. For patients whose primary problems include imbalance, the clinician’s investment of time to perform this comprehensive test battery yields a valuable outcome. Shorter versions of this test, the Mini-BESTest and the Brief-BESTest, have subsequently been published. , They take less time to administer but likewise provide a less complete picture of the patient’s balance systems. Specifically, they do not include any items from the biomechanical constraints or stability limits categories. Even so, they are superior to single-item tests or screening tests that are not based on the systems model and do not identify balance system deficits that should be addressed in the intervention plan.

For some patients at lower functional levels, the BESTest may be too difficult. If this is the case, the Berg Balance Scale (BBS) is also an excellent test battery. Though not specifically designed to align with the systems model of dynamic equilibrium, the BBS contains 14 items that do challenge several different components of postural control. Selection of one of these two test batteries (based on patient ability level) to initiate a comprehensive balance evaluation is a valuable approach because results from these tests permit extensive hypothesis generation about what balance skills may be deficient and what postural control system impairments may be present.
No single, simple test for balance is possible because balance is such a complex sensorimotor process. Many relatively simple balance tests exist, but not all tests are appropriate for all patients. Different tests may be needed to answer specific questions. For example, several good tests have been developed to determine the risk of falls in elderly people. These would be insufficient to discern whether an injured dancer can resume practice, or an injured roofer is ready to return to work. Clinicians should understand the advantages and limitations of different balance tests in order to be able to select appropriate evaluative tools.
In general, a balance test will not be useful unless it sufficiently challenges the postural control system being tested. Tests for stability (“static balance”) are appropriate for patients who are having difficulty simply finding midline or holding still in sitting or standing. They are of much less value for patients with higher-level abilities. Conversely, single-leg stance tests or sensory tests with a foam surface may be far too difficult to perform for patients with lower-level abilities. Certain tests permit the use of arms and/or assistive devices, while others do not; clearly test choices are then limited for patients dependent on the use of upper extremities for balance.
A word of caution about interpreting test results is indicated. Most clinical tests rely on observations of motor behavior to arrive at some conclusion about what systems have problems and how they affect movement. Abnormal motor behavior has many causes, and clinicians should be careful before concluding that an observed behavior is caused by problems in a certain system. For example, the Romberg test is commonly assumed to test the use of vestibular inputs. Yet during the test, both somatosensory and vestibular inputs are (normally) used for balance control. If balance control is deficient, is the vestibular system necessarily the culprit? Could somatosensory system deficits also result in a poor test result? Or, alternatively, because the Romberg test is performed with feet together, what effect would hip weakness have on the ability to stand with a narrowed base of support? When using a test whose results may be altered by problems in more than one system, any relevant system should be evaluated. If multiple system deficits exist, and they often do in patients with neurological conditions, then use caution in making “commonly assumed” conclusions on the basis of clinical test results.
Because so many balance tests are available, several questions must be asked to determine whether a test is appropriate for use. For what purpose and population was the test designed? Can that test be used legitimately for a different purpose or with a different population? Is it valid? Is it reliable, that is, repeatable by different examiners or by the same examiner multiple times? In what populations is it valid and reliable? What is the threshold (minimal detectable change [MDC]) for this test—that is, how large must true performance changes be before this test can detect them? Are normative data available for comparison? These questions are being investigated but have not yet been answered for many of the clinical balance tests commonly used by therapists with the many different neurological populations they treat. Some of the evidence already reported may be frustrating to clinicians. For example, the Timed Up-and-Go test (TUG) predicts falls in community-dwelling older adults but not in acute-care hospital populations. , For several balance tests such as the TUG, the BBS, and others, the cutoff scores used for accurate prediction of falls in patients with Parkinson disease are different from the cutoff scores used in older adults without Parkinson disease. These examples make it clear that clinicians must understand their patients and the characteristics of the various balance tests in order to select the most appropriate tests and interpret test results for each patient.
Types of balance tests
Balance tests can be grouped or classified by type. Different types of tests measure different facets of postural control ( Table 20.1 ). Quiet standing (static) refers to tests in which the patient is standing and the movement goal is to hold still. Disturbances to balance, called perturbations, may or may not be applied. Active standing (dynamic) tests also position the patient standing, but the movement goal involves voluntary weight shifting. Transition tests require that the patient move from one point to another, but do not require walking; examples include items #1 Sit-to-Stand, and #4 Transfer in the BBS, and the 4-Square Step Test (4SST). Sensory manipulation tests use altered surface and visual conditions to determine how well the CNS is using and reweighting sensory inputs for postural control. Functional balance, mobility, and gait scales involve the performance of whole-body movement tasks, such as Item #9 “Pick up object from floor” in the BBS, walking, and stepping over objects. A few test batteries offer a combination of the preceding tests. The BESTest is the most comprehensive test battery to date. Dual-task tests have been developed to examine the effect of concurrent activities and divided attention on balance and mobility performance. Tests of sitting balance in adults are being developed because patients with neurological problems often need sitting balance retraining in early stages of recovery. Examples include the Seated Functional Reach Test (used to measure excursion in seated individuals with spinal cord injuries), Postural Assessment Scale for Stroke Patients (PASS), Trunk Impairment Scale, Kansas University Sitting Balance Scale, Sitting Balance Assessment Tool (SitBAT), Function in Sitting Test, and Ottawa Sitting Scale.
Type | Tests |
---|---|
Quiet standing (with or without perturbation) | Romberg Sharpened Romberg or tandem Romberg One-legged stance test (OLST) Timed stance battery Balance Error Scoring System (BESS) Postural sway via forceplate, accelerometry Nudge or push Push and Release Test Motor Control Test (MCT) |
Active standing | Functional Reach Test (FRT) Multi-Directional Reach Test (MDFR) Limits of Stability (LOS) Star Excursion Balance Test (SEBT) and Y Balance Test |
Sensory manipulation | Sensory Organization Test (SOT) Clinical Test of Sensory Interaction on Balance (CTSIB) |
Active transitions and stepping | 5-Times Sit-to-Stand Test (5XSTS) 30-s Chair Stand Test (CST) 4-Square Step Test (4SST) |
Functional scales | Berg Balance Scale (BBS) Timed Up-and-Go Test (TUG) Tinetti Performance Oriented Mobility Assessment (POMA) (Balance subscale) Tinetti Performance Oriented Mobility Assessment (POMA) (Gait subscale) Gait Abnormality Rating Scale (GARS) Modified Dynamic Gait Index (mDGI) Functional Gait Assessment (FGA) Environmental Analysis of Mobility Questionnaire (EAMQ) |
Combination test batteries | Balance Evaluation Systems Test (BESTest) Fregly-Graybiel Ataxia Test Battery High-level Mobility Assessment Test (HiMAT) Community Balance and Mobility Scale (CBM) Fugl-Meyer Sensorimotor Assessment of Balance Performance |
Dual-task | Stops walking when talking (SWWT) Dual-Task TUG, Manual and Cognitive Multiple Tasks Test (MTT) Walking and Remembering Test (WART) |
Balance confidence | Activities-Specific Balance Confidence Scale (ABC) Falls Efficacy Scale—International (FES-I) Survey of Activity and Fear of Falling in the Elderly (SAFFE) Fear of Falling Avoidance Behavior Questionnaire (FFABQ) |
Quiet standing
The classic Romberg test was originally developed to “examine the effect of posterior column disease upon upright stance.” The patient stands with feet parallel and together and then closes the eyes for 20 to 30 seconds. The examiner subjectively judges the amount of sway. Quantification of sway can be accomplished with a videotape, forceplate, or, more recently, accelerometer. , Excessive sway, loss of balance, or stepping during this test is abnormal. The sharpened Romberg, also known as the tandem Romberg, requires the patient to stand with feet in a heel-to-toe position and arms folded across the chest, eyes closed for 60 seconds. Often four trials of this test are timed with a stopwatch, for a maximum score of 240 seconds.
One-legged stance tests (OLSTs) are commonly used. , Both legs must be alternately tested, and differences between sides are noted. The patient stands on both feet and places hands on the hips or crosses the arms over the chest, then picks up one leg and holds it with the hip in neutral and the knee flexed to 90 degrees. The lifted leg may not be pressed into the stance leg. This test is scored with a stopwatch. Five 30-second trials are performed for each leg (alternating legs), with a maximum possible score of 150 seconds per leg. Normal young subjects are able to stand for 30 seconds, but this may not be a reasonable expectation for frail older patients.
In both the Romberg test and the OLST, problems in sensory organization processes can be observed. To determine how much of the stability is achieved through visual stabilization, each test can be repeated with eyes closed. The patient with visual dependency for balance will often have an immediate loss of balance when the eyes are closed. (Remember, visual dependency may be a sign of somatosensory or vestibular loss, or both.) As noted earlier, the patient with somatosensory or vestibular loss may have difficulty producing the hip strategy necessary to perform these tasks.
A battery of timed stance tests has been developed by Bohannon and Leary ; designed for acute care bedside use, it can be used in other settings as well. This set of tests varies the foot position (apart, together, tandem, and single leg) and the availability of visual information (eyes opened and closed) to produce eight different combinations. Maintenance of balance in each condition is timed for a maximum of 30 seconds; the assigned score is the total number of seconds that balance could be maintained. The best possible score on this test is 240 seconds. This test is reliable, valid, and sensitive to change over time.
A related test is the Balance Error Scoring System, or BESS test, which was developed for use with athletes to screen for concussion effects. , The original BESS test involved three stance positions (double-leg stance with feet together, single-leg stance, tandem stance) on two surfaces (firm and foam), thus providing six conditions. The eyes are closed in all conditions. Each trial is 20 seconds in duration. The examiner observes and counts the number of balance errors that are made in each condition. The six observed errors include hands lifted off waist; opening eyes; step, stumble, or balance loss; moving a hip past 30 degrees of abduction; lifting the forefoot or heel; and remaining out of the test position for more than 5 seconds. If more than one error occurs at the same time, for example, opening eyes and hands off waist, only one error is counted. When measured in high-level young adults, the reliability of this test improved with the removal of the double-leg stance condition, which produced few to no errors in this high-functioning population, and the addition of three trials in each of the remaining four conditions. The modified BESS test thus includes only four conditions. This test has not been investigated for use in traditional neurological rehabilitation populations but may be a valuable alternative for high-functioning young patients post-concussion or mild TBI.
Objective postural sway measures can be obtained by computerized force plates ( Fig. 20.5 ) or wearable accelerometers ( Fig. 20.6 ). , The patient is asked to adopt a standardized foot placement, if possible (this varies by manufacturer), and to stand quietly with arms at the sides or hands on the hips for 20 or 30 seconds. Sway with both eyes open and eyes closed is commonly measured. Graphic and numerical quantification is provided. Normative data may be provided. These more technical measures are able to detect more subtle problems and are more sensitive to change in performance after treatment than are rating scales or timed measures.




APRs are assessed by the patient’s response to perturbations. It is imperative that clinicians include APR testing in their balance assessment because APRs are the motor responses necessary to prevent loss of balance and falls. The push-and-release test is a clinically useful method with a five-point ordinal rating scale. , , Testing for ankle and hip strategies (“in-place” strategies) requires that the clinician (1) place his or her hands on the front or back of the patient’s shoulders, (2) ask the patient to remain still and centered by resisting the pressure applied by the hands (producing isometric muscle activity), (3) watch for the toes or heels to begin to raise slightly (the clinician increases pressure until this occurs), then (4) suddenly release the push. Both forward and backward directions are tested. For safety, the clinician always stands where he or she can support the patient in case of balance loss. Testing for stepping strategy follows the same concept but is performed differently. Instead of keeping the patient’s COG at midline, the patient leans his or her weight into the clinician’s hands, shifting the COG away from midline toward the outer limit of stability before the release. The correct patient response is to step to reestablish a new base of support underneath the new position of the COG. Forward, backward, and both lateral directions are tested. When nudge or push tests are performed predictably (i.e., “Don’t let me push you backward.”), this is assessment of anticipatory postural control. When the release happens unpredictably (no cues, unpredictable timing), APRs can be assessed. Perturbations of different strengths from multiple directions should be given.
The Motor Control Test (MCT) is a computerized test of APRs that perturbs the patient through surface displacement ( Fig. 20.7 B). The patient stands on a dynamic (movable) forceplate with feet parallel and arms at sides. The support surface rapidly translates (slides) forward or backward. This surface displacement results in a rapid shift in the relation between the COG and the base of support. The expected responses are directionally specific (to the direction of the stimulus) forces generated against the surface to bring the COG back to the center. Response latencies, strength, and symmetry are measured. Normative data are available. This test can be used to look for abnormal stepping strategies when failure to select hip strategy occurs. The MCT is the most standardized and reliable test of APRs but is less widely used because it requires computerized equipment.

Active standing
Volitional control of the COG is evaluated by asking the patient to make voluntary movements that require weight shifting. The Functional Reach Test (FRT) was developed for home health use with older adults to determine risk of falls. The patient stands near a wall with feet parallel. Attached to the wall at shoulder height is a yardstick. The patient is asked to make a fist and raise the arm nearest the wall to 90 degrees of shoulder flexion. The examiner notes the position of the fist on the yardstick. The patient is then asked to lean forward as far as possible, and the examiner notes the end position of the fist on the yardstick ( Fig. 20.8 ). Beginning position is subtracted from end position to obtain a change unit in inches. Three trials are performed. Normative data are available, and the test is reliable. However, the standard error of measurement for this test may be as high as 2 inches, meaning that a change in score of less than 2 inches cannot be attributed to clinical improvement because it may reflect only measurement error. Subsequent studies have not shown that this test is useful for fall prediction. For these reasons the FRT is not recommended as a stand-alone test, rather it should be used in conjunction with other tests. Both the BBS and the BESTest include the FRT.

One serious limitation of the FRT is that it measures sway in only one direction (forward). An expansion of this test has been devised to measure sway in four directions. The multidirectional functional reach test (MDFR) is conceptually equivalent but measures sway anteriorly, posteriorly, and laterally to both sides. This test should provide a more comprehensive picture of volitional COG control limitations. Validity and mean values have been established for community-dwelling older adults. The BESTest includes the forward and lateral directions from the MDFR test.
The Limits of Stability test (LOS) uses a computerized forceplate to measure postural sway away from midline in eight directions. , Patients assume a standardized foot position and control a cursor on the computer monitor by shifting their weight. They are asked to move the cursor from midline to eight targets on the screen ( Fig. 20.9 ). Measures include movement velocity, directional control (path sway), measures of excursion (length of the trajectory of the COG), and reaction time. This test should be performed once for familiarization, then a second time for scoring purposes. Second and subsequent tests are reliable and normative data are available.

A very challenging test used primarily in athletic populations is the Star Excursion Balance Test (SEBT) ( Fig. 20.10 ). , The SEBT could be used, for example, in high-level TBI patients who require more demanding test conditions. However, although there is evidence for the validity and reliability of this test in orthopedic populations, as yet this test has not been investigated for use in neurological populations. The SEBT is in concept a lower-extremity functional reach test, requiring single-leg stance on one leg and a reach with the other leg. The original SEBT included eight directions; currently the SEBT is typically performed in three directions: center-forward, right-rear, and left-rear. Three tape measures are taped to the floor, radiating out from the same center point. The two rear tape measures are at a 45-degree angle from the center line. The patient stands on one foot with the great toe on the center point, then reaches the maximum distance away from the center with the lifted foot. The distance is recorded by the examiner. This is done in all three directions, with the lifted leg having to cross behind the stance leg to reach to the opposite-side rear tape. Six practice trials in each direction are given before recording scores to eliminate a learning effect, although recent evidence suggests four practice trials may be sufficient. Three scored trials in each direction are performed; both legs are tested. A commercial version of this test, the Y Balance Test, is available and has the advantage of being portable.

Sensory manipulation
Sensory inputs play a critical role in postural control, but few tests to measure their use to produce a balance performance outcome have been developed. The Sensory Organization Test (SOT) uses a computerized, movable forceplate and movable visual surround to alter the surface and visual environments systematically. , The patient stands on the forceplate with feet parallel and arms at the sides and is asked to stand quietly. Three 20-second trials under each of six sensory conditions are performed ( Fig. 20.11 ). In conditions one, two, and three the support surface (forceplate) is fixed. During conditions four, five, and six the support surface is sway referenced to the sway of the patient. In other words, the movement of the surface is matched to the movement of the patient in a 1:1 ratio. This responsive surface movement maintains a near-constant ankle joint angle despite body sway, rendering the somatosensory information from the feet and ankles inaccurate for use in balance maintenance. Visual inputs are undisturbed in conditions one and four. Vision is absent (eyes are closed) in conditions two and five. The movable visual surround is sway referenced in conditions three and six. This responsive visual surround movement maintains a near-constant distance between the eyes and the visual environment despite body sway, rendering visual inputs from the eyes inaccurate for balance maintenance in those two conditions.

Under condition one, all three senses (vision, vestibular sense, and somatosensory sense) are available and accurate. Body sway is measured by the forceplate; this initial measurement forms the baseline against which subsequent measures are compared ( Fig. 20.12 ). Under condition two the eyes are closed, so only somatosensory and vestibular cues remain. In an individual with normal movement function, the somatosensory inputs will dominate in this condition. By comparing sway during condition two with sway during condition one, detection of how well the patient is using somatosensory inputs for balance control is possible. Patients with somatosensory loss from incomplete SCI, diabetes, or amputation have difficulty in condition two. Functional situations with inadequate lighting or unusable visual cues (e.g., busy carpeting) are similar to condition two.

Under condition four, the support surface is sway referenced (somatosensory cues are available but are inaccurate), so only visual and vestibular cues remain useful. In a normal patient the visual inputs will dominate in this condition. Comparing sway during condition four with sway during condition one indicates how well the patient is using visual inputs for balance control. Patients with visual loss caused by diabetes, cataracts, or field loss have difficulty in condition four. Functional situations that correlate with condition four include compliant surfaces (beach, soft ground, gravel driveway) and unstable surfaces (boat deck, slipping throw rug).
Under condition five, the eyes are closed (visual cues are absent) and the support surface is sway referenced (somatosensory cues are inaccurate), leaving the vestibular inputs as the only remaining sensory inputs that are both available and accurate. Comparison of sway during condition five with sway during condition one indicates how well the patient is using vestibular inputs for balance control. Patients with vestibular loss caused by head injury, MS, or acoustic neuroma may have difficulty with condition five. Many older patients with age-related vestibular loss also may be unstable in this condition. Functional situations in which these patients may be at risk for falls would have both inadequate lighting and compliant or unsteady surfaces (e.g., walking on a gravel driveway or thick carpet in the dark).
Under both conditions three and six, the visual surround is sway referenced (visual cues are available but inaccurate). By comparing sway during these two conditions with sway in the absence of vision (conditions two and five, with eyes closed), determining how well the patient can recognize and subsequently suppress inaccurate visual inputs when they conflict with somatosensory and vestibular cues is possible. Some patients with CNS lesions (e.g., head injury, stroke, tumor) may have difficulty with this condition. Patients who cannot recognize or ignore inaccurate visual cues cannot distinguish whether they are moving, or the environment is moving. If they perceive that they are moving (away from midline) when they are not, they may often actively generate postural responses to “right” themselves. These responses, invoked to bring the COG to midline, then result in movement away from the midline. The inaccurate perception leads to a self-initiated loss of balance. Functional situations that correlate with this test condition include public transportation, grocery and library aisles, and moving walkways.
The SOT is valid and reliable in the absence of motoric problems, which increase sway for reasons unrelated to sensory reception and perception. Normative data are available.
The Clinical Test of Sensory Interaction on Balance (CTSIB) is a clinical version of the SOT that does not use computerized forceplate technology. The concept of the six conditions remains intact ( Fig. 20.13 ). Instead of sway measures, the examiner uses a stopwatch and visual observation. A thick foam pad substitutes for the moving forceplate during conditions four, five, and six. In normal individuals and patients with peripheral vestibular lesions, measures with foam correlate to moving forceplate measures. Originally, a modified Japanese lantern substituted for the moving visual surround in conditions three and six. Studies have not shown that measures using the Japanese lantern correlate with the moving visual surround measures. Most clinicians now perform the modified CTSIB with just four conditions, eyes open and closed on a firm surface and eyes open and closed on the foam surface. The patient is asked to stand with feet parallel and arms at the sides or hands on the hips. At least three and up to five 30-second trials of each condition are performed. The watch is stopped if the patient steps, reaches, or falls during the 30 seconds. If the patient is very steady for 30 seconds on the first trial of a condition, some clinicians choose not to test the remaining trials in that condition and will give the patient a full score for that condition. A maximum score for five trials of each condition is 150 seconds. Individuals with normal movement abilities are able to stand without stepping, reaching, or exhibiting loss of balance for 30 seconds per trial per condition. It is normal for sway to increase slightly as the conditions increase in difficulty. The CTSIB may not be a reliable measure in patients with hemiplegia or other conditions that involve motor deficits in, or abnormal response time through, the lower extremities and trunk. The clinician can use the information regarding patient response in a variety of environmental conditions to determine intervention management strategies.

Vestibular system tests
Please refer to Chapter 21 for a thorough presentation of vestibular disorders and their management.
Active transitions and stepping
The ability to change the base of support without balance loss and then to reestablish COG stability over the new base of support is a balance-dependent skill critical for functional activities. The 5-Times Sit-to-Stand Test (5XSTS) and the 30-second Chair Stand Test (CST) are both quantifiable measures of the sit-to-stand skill often used to screen for leg weakness. , For both tests, the patient sits with arms crossed over chest in a stabilized standard height chair without arms. The starting position is seated slightly forward so that the patient is not resting against the back of the chair, with the feet underneath and slightly behind the knees. In the 5XSTS the patient is asked to stand up (all the way up to an erect stance) and sit all the way back down (weight transferred from feet to buttocks) 5 times; the clinician uses a stopwatch to time the performance beginning when the clinician says “Go” and ending when the patient is fully seated the fifth time. In the CST the instructions to the patient and their performance of full sit-to-stand motions are the same, but the clinician counts the number of times the patient can perform the motion in a 30-second timeframe. Rests are permitted but the timer continues to run, so rests should only be taken if needed. The longer duration of this test requires muscle endurance in addition to strength. Normative data and cutoff points for fall risk screening are available for both tests.
The 4-Square Step Test is a timed stepping test in a standardized, structured format in forward, backward, and lateral directions ( Fig. 20.14 ). A simple plus sign–shaped grid is laid out on the floor using four straight canes, dowel rods, or lengths of plastic piping. This creates four quadrants. The patient begins standing in the rear-left quadrant, steps forward over the first bar to stand with both feet in the front-left quadrant, steps rightward over the second bar to stand with both feet in the right-front quadrant, steps backward over the third bar to stand with both feet in the right-rear quadrant, steps leftward over the fourth bar to stand with both feet in the left-rear quadrant (starting location). The patient then reverses direction, going back through each quadrant in the same way until standing again with both feet in the rear-left quadrant. The outcome measure is the time it takes the patient to perform this task correctly, clearing each bar completely with each foot. This test has been used with older adults, individuals with vestibular disorders, and with patients post-stroke. ,

Functional scales
A comprehensive balance evaluation must include impairment-based tests of body systems, tests of balance skills, and activity-based functional measures. Functional scales help identify the activity limitations. By asking the patient to perform functional tasks that demand balance skills, the clinician can determine the presence of activity limitations that will affect the individual’s ability to participate in life and identify the tasks that the patient needs to practice. Three mobility scales and three gait scales focus on postural control; five of these were developed for the elderly population to determine risk of falls. Many clinicians are also using them to assess patients with neurological conditions, although their usefulness with neurological populations is less well-documented. Far fewer standardized tests for high-level balance skills have been developed; some clinicians adapt tests used by athletes, but these are often too difficult for many neurologically involved patients.
The BBS consists of 14 tasks that the patient is asked to perform. The examiner rates the patient on each task by using an ordinal rating scale of 0 to 4, in which 0 is unable to perform and 4 is able to perform without difficulty. This test is highly reliable. It was originally designed for assessing risk of falls in older adults, and cutoff scores for fall risk vary depending on which of several studies is consulted. , Use of higher cutoff scores may erroneously identify nonfallers as fallers; use of lower cutoff scores may erroneously identify fallers as nonfallers. The BBS has also been used with patients after stroke.
The original Get-Up-and-Go Test is made up of seven items and subjectively scored on an ordinal rating scale of 1 to 5, in which 1 is normal and 5 is severely abnormal. This test has been modified by making it a timed measure to increase its objectivity and reliability, which is now high. The TUG test eliminates the “standing steady” segment and uses a stopwatch to time the performance. Patients are asked to rise from a chair with arms, walk 3 meters as fast as they safely can, turn, walk back to the chair, and sit down. This test may be performed with an assistive device; however, the use of a device will alter the speed at which the task can be accomplished, and any retesting must be done with the same device to produce comparable results. Originally designed to assess frailty in older adults, the test is now more commonly used to assess fall risk in this population. Young adults typically perform this task in 5 to 7 seconds, healthy older adults in 7 to 9 seconds (low-risk), moderate-risk older adults in 10 to 12 seconds, and high-risk older adults in 13 seconds or more. , These cutoff scores are for older adults walking without assistive devices. Improvements in test performance that are not captured by the time score alone should also be documented; for example, if the patient can now perform the test without the use of chair arms to stand up, or without an assistive device.
The Tinetti Performance-Oriented Mobility Assessment—Balance subscale (POMA-Balance) is a list of nine items scored on scales of either 0 to 1 or 0 to 2, with the higher numbers reflecting better (more normal) performance. The score value is specific to the item. The best possible score is 16, with a score of 10 or lower indicating a high risk of falls. This test is appropriate for onetime use, for example at a health fair, but is not ideal for documenting progress over time due to the limited scoring range.
Most balance and mobility scales have been developed to assess risk of falls in older adults. Many share similar items. See Table 20.2 for a summary of scale items.
Activity | Berg Balance Scale | Dynamic Gait Index and Functional Gait Assessment | Timed Up-And-Go Test | Tinetti Balance Assessment |
---|---|---|---|---|
| ✓ | ✓ | ||
| ✓ | ✓ | ✓ | |
| ✓ | ✓ | ✓ | |
| ✓ | |||
| ✓ | ✓ | ✓ | |
| ✓ | ✓ | ||
| ✓ | |||
| ✓ | |||
| ✓ | |||
| ✓ | |||
| ✓ | |||
| ✓ | ✓ | ||
| ✓ | |||
| ✓ | |||
| ✓ | ✓ | ||
| ✓ | ✓ | ||
| ✓ | |||
| ✓ | ✓ | ||
| ✓ | |||
| ✓ | |||
| ✓ | |||
| ✓ | |||
| mDGI only | |||
| FGA only | |||
| FGA only | |||
| FGA only |
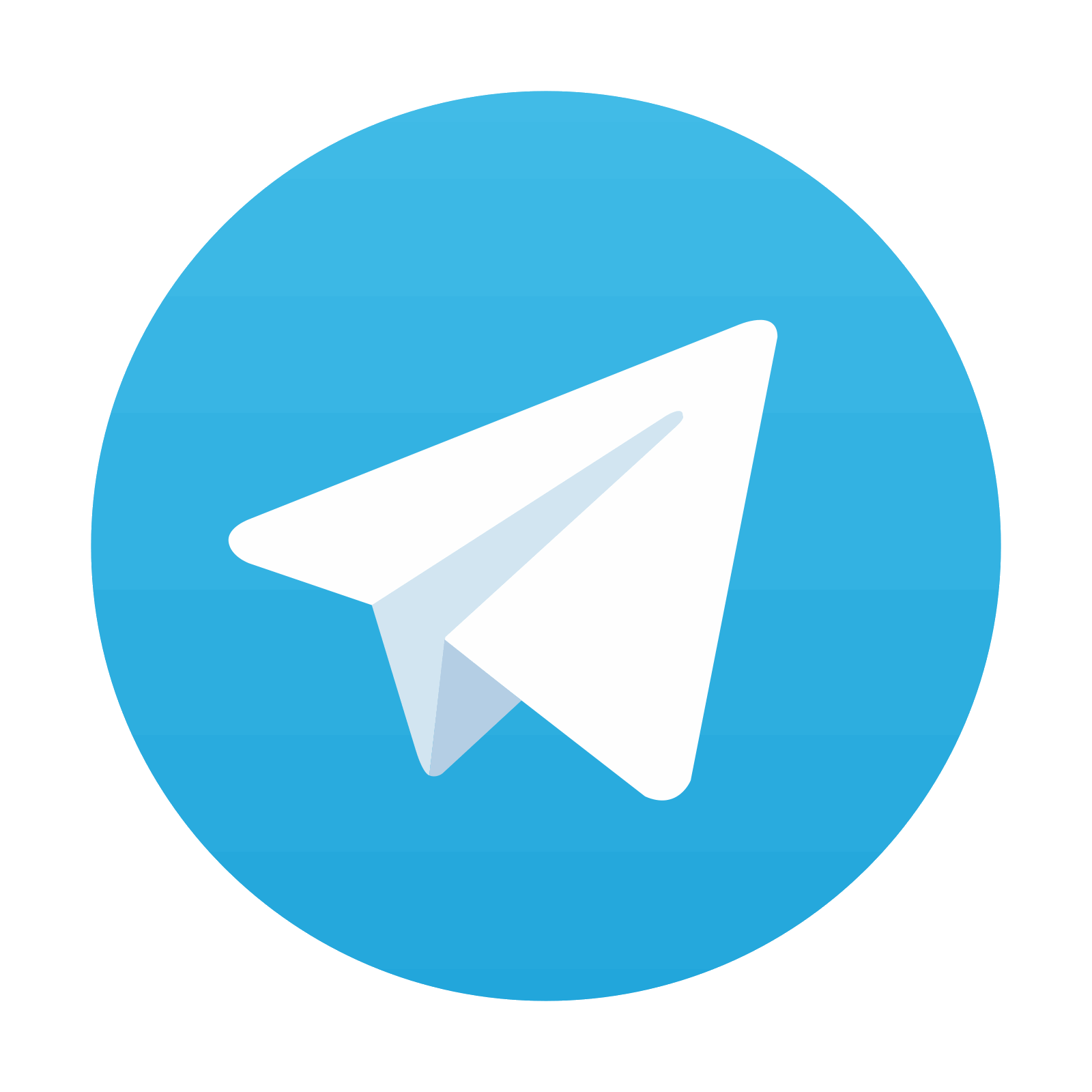
Stay updated, free articles. Join our Telegram channel
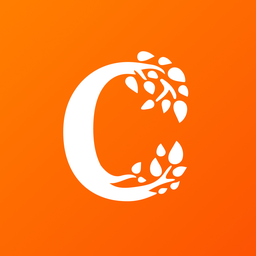
Full access? Get Clinical Tree
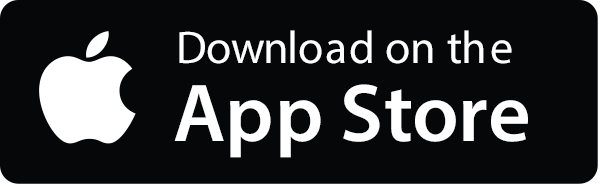
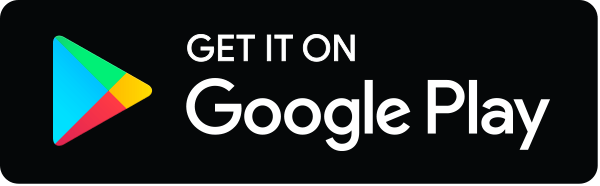
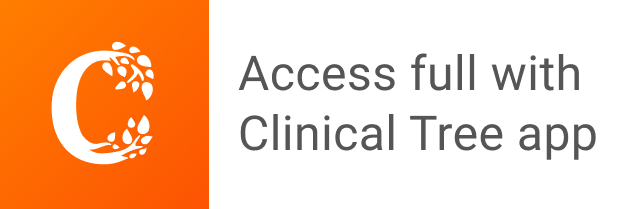