Basic Neuroscience of EEG
L. John Greenfield Jr
DISCOVERY OF CEREBRAL POTENTIALS AND DEVELOPMENT OF EEG
Electricity in the Brain
We take for granted that the brain is the source or our thoughts and actions and that these behaviors arise from electrical impulses generated in neurons, conducted down axons, and transmitted across synapses to other nerve and muscle cells. But the discovery of the electrical nature of nerve cell activity is relatively recent. The idea that the brain controls the muscles by electrical impulses arose in the early 18th century. One of the early proponents was Isaac Newton, who wrote in the Opticks (1717):
Is not animal motion performed by the vibrations of this medium [electrical fluid] excited in the brain by the power of the will, and propagated from thence through the solid pellucid and uniform capillamenta [axons] of the nerves into the muscles, for contracting and dilating them?1
Newton’s hypothesis was based on new evidence linking physical forces with biological functions. William Gilbert had described his experiments with electricity and magnetism in De Magnete (1600), and there was a growing appreciation of electricity as a mysterious force that might be the “animal spirit” secreted by the brain to control movement, sensation, and thought.
The Age of the Electricians
In the 18th century, electricity was all the rage. Static electricity had been known since ancient Greece, when Thales of Miletus noted that a piece of amber rubbed on animal fur would pick up small dust particles or a feather. But friction-generated electricity had its limitations: it was unpredictable, and there was no way to store or measure the charge. Technologic advancements like the invention of the Leyden jar in 1745 by the Dutch scientist, Pieter van Musschenbroek, allowed electrical charges to be stored and later discharged, which enabled the study of electricity under controlled conditions. Public displays of electricity were popular entertainment, such as sending shocks through lines of unwary volunteers. The most famous of the “electricians” was Benjamin Franklin (1706-1790), among whose accomplishments were the invention of the lightening rod and the concept that positive and negative charges were just two states of the same “fluid,” which was present in all things. His famous 1752 experiment2 demonstrating that lightening was caused by electricity in the atmosphere resulted in the deaths of a few other would-be scientists who tried to reproduce his findings. Franklin’s kite was not actually struck by lightning, which might have electrocuted him. He took the precaution of standing inside the open door of a shed and holding a dry silk thread tied to the hemp kite string as an insulator. The kite collected electrical charge from the cloud, conducted it down the wet string, and transmitted it via a brass key into a Leyden jar for later experiments, demonstrating that atmospheric electricity was the same as that obtained by rubbing a glass rod with silk cloth or any other method of generating static electricity. This experiment cemented his fame and led to awards including the Copley Medal in 1753 and membership in the Royal Society in London.
REVIEW
1.1: Who postulated that the brain uses electricity to communicate with the muscles?
1.2: What 18th-century invention allowed storage of electricity for later experiments?
Galvani, Ampere, Kelvin, and the Galvanometer
Experimental support for the idea that the brain controls the muscles by means of electricity came in the 1770s, when Luigi Galvani (1734-1797) applied electrical shocks to frogs’ legs, causing them to twitch. Galvani also found that nerve impulses could pass through metal to activate a muscle. This led him to conclude that “animal electricity” produced by the nerves is the signal for muscular contraction. How could this current be measured?
In 1820, Hans Christian Oersted (1777-1851) discovered that electric current flowing in a wire created a magnetic field around it, which would deflect a magnetized needle. By coiling a length of wire and using the magnetic field generated within the coil to move the magnetized needle proportionately to the amount of current flowing, André Ampère (1775-1836) used this effect to measure electric current. He named the device a “galvanometer” in honor of Galvani, and the unit of current measured was subsequently named for Ampère. The galvanometer rapidly underwent a series of refinements. Rather than a moving magnetized needle, a moving coil of finely wound wire was attached to a pointer and mounted between the poles of a fixed magnet. When electricity passed through the coil, the pointer would deflect proportionally to the amount of current along a graduated scale. In 1858, William Thomson, Lord Kelvin (1824-1907), invented a mirror galvanometer, which was used to measure the electric current passing through the transatlantic telegraph cable constructed between the United States and Europe. A mirror was attached to the coil, on which a light was shined. Electric current moved the coil, and the deflection of the light was measured on a scale, amplifying the sensitivity to small deflections. Such a device could also be used to measure the tiny electrical currents generated in the brain.
In 1867, Thompson further refined the galvanometer by inventing the “siphon recorder,” in which ink was siphoned by capillary action through a thin glass tube attached to the wire coil, mounted between the poles of a magnet. The moving tube carried the ink onto a paper tape, where it traced a line. The chart recorder was born and remained in use for electroencephalography (EEG) recordings well into the 1990s when it was replaced by digital recording systems. Even today, pen-and-ink chart recorders are still used by some centers for EEG recordings, and old-school EEG technologists reminisce about the intricacies of these devices.
REVIEW
1.4: Who refined the galvanometer to make possible moving pen chart recorders?
Caton, Berger, and the Discovery of EEG
Richard Caton (1842-1926) was the first physiologist to record spontaneous electrical activity in the mammalian brain, using the dog as a model.3 In 1875, he wrote:
In every brain hitherto examined, the galvanometer has indicated the existence of electric currents. The external surface of the grey matter is usually positive in relation to the surface of a section through it. Feeble currents of varying direction pass through the multiplier [amplifier] when the electrodes are placed on two points of the external surface of the skull.4
Caton used the mirror galvanometer to amplify the miniscule voltage signal. He was the first to find that interrupting the light transmitted to a dog’s eye altered the electrical waves detected from the opposite side of the brain (Fig. 1.1).
In 1924, Hans Berger (1873-1941) obtained the first recordings of brain electrical potentials in humans. His experiments were motivated by a belief that electrical activity in the brain could be a mechanism underlying telepathy. When he was a young soldier serving in the Austrian Cavalry, his horse reared and threw him in the path of a horse-drawn cannon, which fortunately stopped in time to
prevent him from being injured. At the same time, his sister at home had a feeling he was in danger. He later wrote, “It was a case of spontaneous telepathy in which at a time of mortal danger and as I contemplated certain death, I transmitted my thoughts, while my sister, who was particularly close to me, acted as the receiver.”5 Berger was convinced that there was a physiological cause of this “psychic energy.” He studied medicine, became a psychiatrist, and experimented with human EEG recordings using scalp surface electrodes, initially in World War I soldiers with underlying skull defects and later through the intact cranium. He found that the best recordings were obtained with an electrode on the occipital head region and a second electrode on the frontal region. In a 1929 paper,6 he published the first EEG recordings from humans, noting that:
prevent him from being injured. At the same time, his sister at home had a feeling he was in danger. He later wrote, “It was a case of spontaneous telepathy in which at a time of mortal danger and as I contemplated certain death, I transmitted my thoughts, while my sister, who was particularly close to me, acted as the receiver.”5 Berger was convinced that there was a physiological cause of this “psychic energy.” He studied medicine, became a psychiatrist, and experimented with human EEG recordings using scalp surface electrodes, initially in World War I soldiers with underlying skull defects and later through the intact cranium. He found that the best recordings were obtained with an electrode on the occipital head region and a second electrode on the frontal region. In a 1929 paper,6 he published the first EEG recordings from humans, noting that:
The electroencephalogram represents a continuous curve with continuous oscillations in which … one can distinguish larger first order waves with an average duration of 90 milliseconds [alpha waves] and smaller second order waves of an average duration of 35 milliseconds [beta waves]. The larger deflections measure at most 150 to 200 microvolts….
Berger made a number of additional discoveries, including the loss of alpha activity in sleep and with eye opening, as well as brain wave changes associated with brain tumors and epilepsy. These groundbreaking findings made him the father of human EEG. His results were initially disbelieved and ignored by the scientific community, but the cerebral origin of EEG waves was subsequently confirmed by the English physiologists Edgar Adrian and B.C.H. Matthews,7 and by the mid-1930s, he was recognized internationally for his achievements. In Germany, however, his work was not appreciated, and his distaste for the Nazi Party led to his forced retirement in 1938. After a long depression, he committed suicide in 1941.
REVIEW
BASIC PRINCIPLES OF ELECTRICITY
Electricity Is the Movement of Charged Particles
We usually think about electricity as moving through wires, but of course, lightening is the movement of charged particles through the air, and shuffling your feet across the carpet in winter can cause a nasty shock when you reach for the doorknob. The discharges created by static electricity or lightening, like those passing through a wire, are due to the movement of electrons, but in the brain, the charges are carried by sodium, potassium, calcium, or chloride ions passing through ion channels or tissues. In either case, the same electrical principles apply. Movement of charged particles is defined as an electric current (I), which is measured in amperes (A). One ampere is the movement of one coulomb (Q; the standard unit of charge, about 6.24 × 1018 charged particles) over 1 second or about the amount of current that flows through a standard 100-watt light bulb. Conversely, the elementary charge of a proton was recently redefined as 1.602 × 10-19 coulombs. Charges move because they are driven by electromotive force, or voltage (V), that results from a difference in electrical potential between two places. Current flows from a region of higher potential to one of lower potential, impeded by the resistance of the conductor, measured in ohms (Ω). Alternatively, one can think in terms of conductance (g), which is the inverse of resistance and measured in units called Siemens (where S = 1/Ω). The resistance to current flow is defined by the amount of current that can flow against it when driven by a given voltage. This fundamental relationship is Ohm law:
Voltage = Current × Resistance, or V = I × R
Or alternatively,
Voltage = Current/Conductance, or V = I/g
It is easier to visualize the roles of voltage, current, and resistance if we use the analogy of water flowing through pipes (Fig. 1.2A). The voltage source (a battery or a power supply) can be imagined as a pump that pushes water to a higher level, where it has the potential energy to flow downward due to gravity and do work. Voltage is like the pressure that lifts the water. After being lifted to a higher potential, the water flows down toward ground level through pipes, some narrower (higher resistance) than others. Like ground level for water, electrical ground is the lowest point in the circuit, where charges have no residual potential energy or ability to do work. Another key concept is capacitance, the ability to store electrical energy, which in the water analogy is equivalent to a storage tank (Fig. 1.2A). Unlike the water tank, however, electrical charge is stored most efficiently when conductors are separated by a very thin nonconducting (dielectric or insulating) substance, allowing opposite charges to line up along either side. Capacitance increases proportional to the surface area of the conductor plates and inversely proportional to the separation distance between the plates. Capacitance, measured in farads (F), is defined as the amount of charge stored per unit of voltage, or C = Q/V. Another parameter, inductance, results from the generation of a magnetic field by current flowing through a wire, but this only becomes significant when long stretches of wire are coiled together so that the magnetic fields overlap. Inductance is what makes the coil of the galvanometer deflect when current passes through it and is also the force that
powers electric motors, relays, solenoids, and other devices. Since there is no common biological equivalent to an inductor, we will ignore it (for now).
powers electric motors, relays, solenoids, and other devices. Since there is no common biological equivalent to an inductor, we will ignore it (for now).
A few other terms should be defined here. When we lift a bucket of water to a higher level, we are doing work on the water. Lifting it to a height gives the water potential energy, which can be used to drive a waterwheel (Fig. 1.2A). Similarly, when a charged particle is moved by electromotive force (voltage), we can say that work has been done on that particle, which now has the potential energy to drive a motor (Fig. 1.2A). Work is thus defined as charge times voltage, or W = Q × V, which is expressed in joules (J). One joule is the work required to move 1 coulomb of charge across a potential difference of 1 volt. The rate of doing work is called
power or energy, expressed in joules/second or watts. A watt is the energy required to move 1 coulomb of charge across 1 volt of potential difference in 1 second. Since charge flowing per unit of time is current, power is thus equal to voltage times current (P = I × V). Substituting terms using Ohm law, it can also be written as P = I2 × R or, alternatively, P = V2/R.
power or energy, expressed in joules/second or watts. A watt is the energy required to move 1 coulomb of charge across 1 volt of potential difference in 1 second. Since charge flowing per unit of time is current, power is thus equal to voltage times current (P = I × V). Substituting terms using Ohm law, it can also be written as P = I2 × R or, alternatively, P = V2/R.
While these equations do not come up very often in our routine consideration of EEG recordings, we sometimes talk about which frequencies are most prominent in a recording in terms of the energy or power in that band (range) of frequencies.
It turns out that biological membranes are composed of elements that nicely correspond to resistors, capacitors, batteries, and other electrical devices (Fig. 1.2B). Ion transporters like the sodium/potassium ATPase that moves 3 Na+ ions outward and 2 K+ ions inward, driven by the hydrolysis of ATP, create an electrochemical gradient (potential difference or voltage) across the plasma membrane that functions as a capacitor. From an electrical perspective, the Na+/K+ ATPase is like a battery creating the charge differential that runs the circuit, but it can also be viewed as a motor that runs on ATP. The narrow lipid bilayer of the plasma membrane (about 7.5 nm thick) functions as a capacitor, with charged ions lining up on either side. Each square centimeter of membrane corresponds to about 1 µF of capacitance. Figure 1.2C shows an equivalent circuit for the cellular circuit elements depicted in Figure 1.2B.
Neurotransmitter Receptors and Channels
Ion channels that span the membrane function as conductors of ionic current, which makes them resistors, since conductance (g) is the inverse of resistance (R). When channels open, charged ions flow down their electrochemical gradients to generate synaptic or action potential currents. Such channels come in two main categories, those that open in response to a change in voltage (voltage-gated channels) and those that open after binding a neurotransmitter molecule (ligand- or receptorgated channels). Each of these types has a variety of flavors. The ligand-gated ion channels that open after binding glutamate (which are named for more specific ligands at the glutamate binding site: AMPA [α-amino-3-hydroxy-5-methyl-4-isoxazolepropionic acid], NMDA [N-methyl-D-aspartate], and kainate receptors) mediate excitatory synaptic transmission by conducting sodium and sometimes calcium ions. Inhibitory ligand-gated channels opened by GABA or glycine conduct chloride ions, usually resulting in inhibition of neuronal firing. Despite conducting positively charged ions, voltage-gated potassium channels tend to be inhibitory, since the concentration of potassium is higher inside the cell than out. When K+ channels open in response to a gradual depolarization or an action potential, K+ ions flow outward, resulting in hyperpolarization of the cell membrane. Voltage-gated sodium, potassium, and calcium channels generate action potentials that mediate long-range transmission of neuronal signaling down axons from one neuron or one brain region to another.
Most neuronal electrical activity is initiated by chemical neurotransmission at synapses, producing excitatory or inhibitory synaptic potentials. Excitatory postsynaptic potentials (EPSPs) elicited by presynaptic release of glutamate have a rise time and decay that depends on which type of postsynaptic glutamate receptors are activated. Depolarizing currents mediated by postsynaptic AMPA and kainic acid receptors have very rapid rise and then decay over 10-25 ms, while those mediated by NMDA receptors have slower rise time (15-25 ms) and longer decay (75-100 ms). NMDA receptors, which conduct both Na+ and Ca2+ ions, are blocked by Mg2+ ions unless the postsynaptic cell is depolarized, usually by prior or simultaneous activation of AMPA receptors. NMDA receptors are critical for strengthening coactivated synapses by Ca2+-dependent mobilization of intracellular AMPA receptors to the cell surface, using a form of synaptic plasticity known as long-term potentiation (LTP). LTP is an important mechanism for stabilizing and strengthening synaptic pathways involved in learning and memory and likely participates as well in strengthening abnormal synaptic activity involved in epileptogenesis. Additionally, three families of metabotropic (G-protein-mediated) glutamate receptors have modulatory effects that can increase or decrease neuronal excitability by interacting with K+ or Ca++ channels or intracellular signaling mechanisms. Inhibitory postsynaptic potentials (IPSPs) generated by the presynaptic release of gamma-aminobutyric acid (GABA) have very rapid rise time (under 1 ms) and decay (tens of ms) when mediated by ionotropic GABAA receptors, but are slower in onset and last up to 150 ms when mediated by metabotropic GABAB receptors. Inhibition can also be mediated by activation of voltage- or second messenger-gated potassium channels.
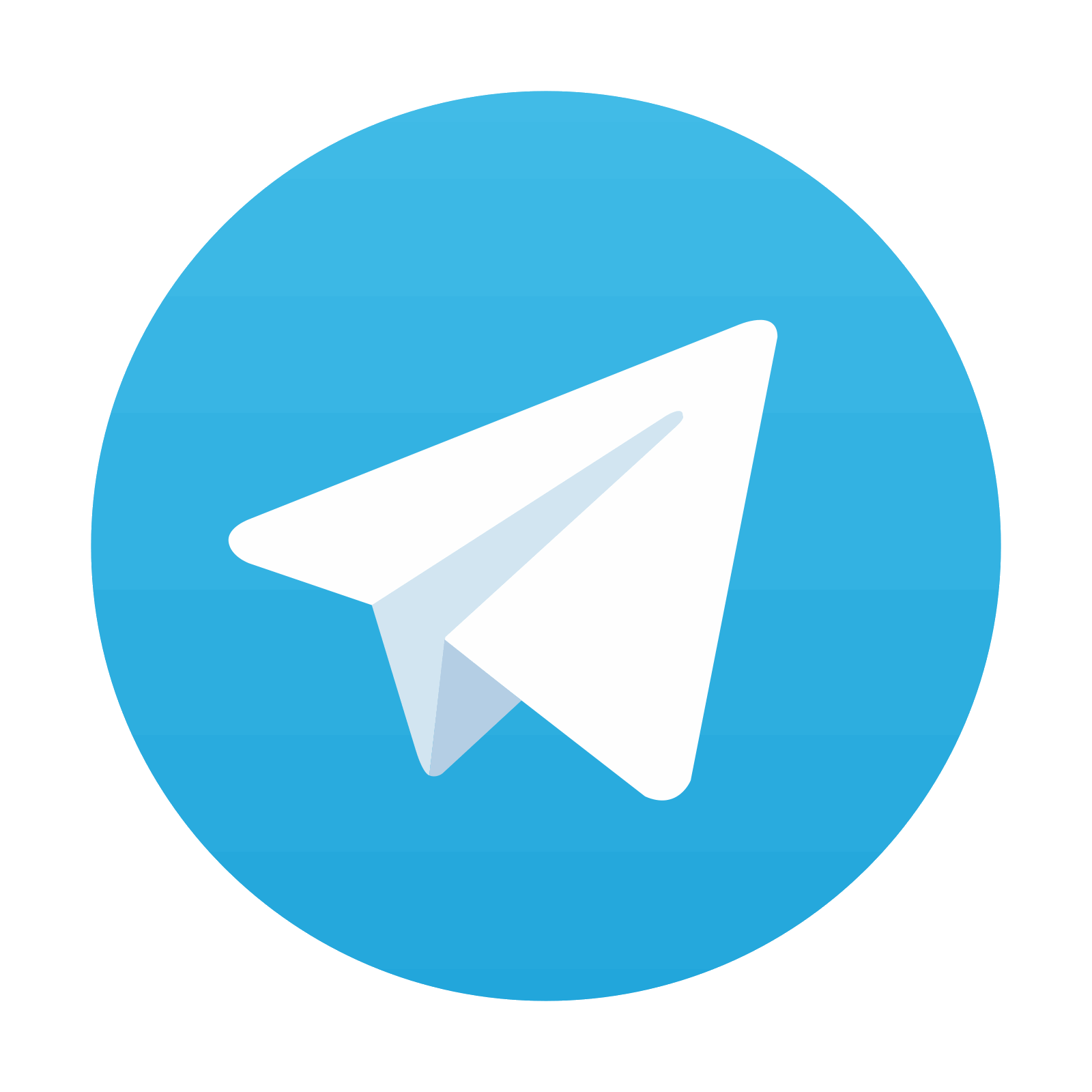
Stay updated, free articles. Join our Telegram channel
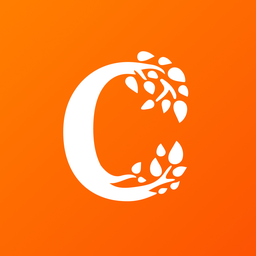
Full access? Get Clinical Tree
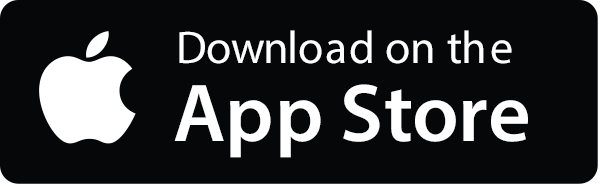
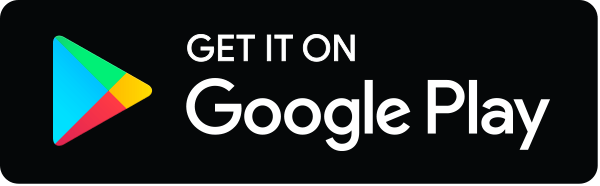