11 Basics of EEG Monitoring in Pediatric Critical Care LEARNING OBJECTIVES • To review basic principles of EEG • To recognize features of the normal pediatric EEG • To understand the basic principles of referential and bipolar montages • To identify EEG patterns specific to ICU recordings Introduction Since the first recording of the electroencephalogram in humans by Hans Berger nearly 100 years ago, EEG has been used in an increasingly sophisticated fashion as a noninvasive means to identify seizures, to localize and assess cerebral dysfunction, and to aid in determining neurologic prognosis.1 Over the past decade, the use of EEG in the pediatric ICU has expanded rapidly.2,3 This chapter provides an overview of the basic principles of EEG monitoring with an emphasis on ICU recordings. In order to appreciate abnormalities that appear in the EEG tracing, it is important to be familiar with the normal patterns of infancy, childhood, and adolescence. The EEG of term and preterm neonates changes dramatically according to gestational age and is outside the scope of this discussion. Basic Principles of EEG Relevant to the ICU Basic Neuronal and Synaptic Physiology The billions of neurons in the human brain communicate via electrical signaling, and it is the composite of these electrical signals that is captured on the surface EEG. It has been estimated that scalp electrodes detect the activity of an approximately 6 cm2 area of underlying cortex, with the ability of each electrode to detect electrical activity dependent on the intensity of the activity and its distance from the cortical surface. Of note, the activity generated by cortical wave generators located deep in the brain may be indirectly measured by scalp electrodes given that they influence neurons on the cortical surface, which are preferentially recorded by scalp EEG.4 EEG discharges are either surface-negative or surface-positive in polarity, and the epileptic spike, which tends to be scalp-negative, is a useful example for understanding polarity. Consider first that Na+/K+ pumps in the cell membrane constantly pump three Na+ ions out of the cell for every two K+ ions pumped into the cell. This results in the establishment of the well-known transmembrane gradient—Na+ is more plentiful outside of the cell while K+ is more plentiful inside the cell. This 3-for-2 exchange of cations leaves a positive charge on the extracellular membrane and a negative charge on the intracellular membrane. Neuronal excitation is a reversal of this polarized negative intracellular and positive extracellular resting state. For example, opening of Na+ or Ca++ channels prompts an inflow of cations into the cell along both charge and concentration gradients, resulting in depolarization of the membrane; this can trigger an action potential if the magnitude of the depolarization is sufficient. Excitatory neurotransmitters (e.g., glutamate) function in this manner, opening channels that allow cations into the nerve cell, thereby causing the membrane to depolarize. The depolarization that these neurotransmitters create is called an excitatory postsynaptic potential (EPSP). Conversely, inhibitory neurotransmitters (e.g., gamma-aminobutyric acid [GABA]) open channels that allow Cl− ions to flow into the cell, thus increasing the baseline intracellular negativity. This causes a hyperpolarization that makes the inside of the cell membrane even more negative and is called an inhibitory postsynaptic potential (IPSP).4,5 Epileptic spikes represent sudden, synchronous excitatory inputs on a group of pyramidal cell bodies. Up to now, we have been observing these charge shifts from perspectives both inside and outside of the neuron, but now let’s consider the EEG electrode’s point of view, which observes extracellular voltage changes from its perch on the scalp. As mentioned, EPSPs open cation channels and facilitate the flow of positively charged ions away from the scalp electrode—they can be thought of as disappearing into the neuron’s intracellular space. This disappearance of positivity is sensed by the electrode as a negative voltage event. Indeed, the inward shift of cations is balanced by compensatory ion shifts deeper in cortex so the net charge remains more or less steady, but because these compensatory shifts occur deeper in cortex, they are not as well “seen” by the EEG electrode. For this reason, the large majority of epileptic spikes recorded at the scalp show negative polarity.4 Thus, the EEG waves we record at the scalp represent the summation of the EPSPs and IPSPs that impinge on neurons at the cortical surface. Different regulators of these cortical potentials, including thalamocortical projections, can lead to neuronal synchronization and the formation of a variety of both normal and epileptiform rhythms.4,5 2Electrode Types and Imaging Compatibility Most EEG electrodes are cup-shaped and are applied to the scalp using an electrolyte paste. The electrode is made of a metal that shares a common ion with the paste so as to allow the free flow of anions; most commonly, silver-coated silver chloride electrodes are used. The skin in the area of electrode placement is prepared prior to application by removing dead skin cells and oils in order to decrease electrode impedance.6,7 Critically ill patients in the ICU often require anticipated and unanticipated neuroimaging studies. Standard metal electrodes are not compatible with MRI scanners and create significant artifact when used in conjunction with CT scanning. Removal and replacement of the electrode set for imaging may be time-consuming and upsetting for the conscious patient. MRI-compatible electrodes crafted from silver, nylon, and Teflon may be used,8 with the caveat that some electrode positions (e.g., certain references, electromyography [EMG], and EKG leads) may need to be eliminated if those electrodes are not safety-approved for the MRI-compatible electrode set. Materials for such electrode systems must be chosen such that the electrode and attached wire will not heat up in the MRI’s magnetic field or disturb image production. Similar plastic electrodes are also available that are CT-compatible and create minimal artifact.9 Because EEG recordings in the ICU are often prolonged, regular surveillance for skin breakdown at the electrode sites should be performed according to a set protocol. At our institution, we use the following schedule for skin checks: An EEG technologist checks scalp skin every 24 hours during continuous EEG (cEEG) monitoring, particularly under Fp1, Fp2, F7, F8, O1, and O2, the electrode positions under which skin is most susceptible to breakdown. If skin irritation is found, affected leads are moved to neighboring 10-10 locations (see below). For example, if there is irritation at Fp1 and Fp2, these leads are moved to the AF3 and AF4 positions. A new montage is created with the relocated leads. Choice of Montage 10-20 System and Types of Montages Electrodes are applied according to the International 10-20 system, a system for measuring and naming EEG electrode positions.10 The name “10-20” is derived from the strategy of making initial standard head measurements, then placing electrodes at intervals of 10% or 20% between those standard landmarks (Figure 1.1).11 Once 19 or more EEG electrodes have been placed according to the 10-20 system, pairings of electrodes are chosen for display, and each such pairing (e.g., Fp1–F3) is referred to as a derivation. The voltage difference between the two electrodes in each derivation is displayed on the screen as an EEG channel and constitutes the essential element of the electroencephalogram. The two electrode inputs of any channel (e.g., Fp1 and F3) are attached to the two poles of a common mode rejection amplifier (CMR). The CMR amplifier allows for the second input to be subtracted from the first input such that any noise signal common to both channels is canceled out. The difference signal (whatever is different between inputs 1 and 2) is amplified. Without use of the CMR amplifier, the environmental noise that runs through the body would swamp the low-voltage EEG signal from the brain, making the tracings uninterpretable. The individual channels are then arranged as a montage, a specific, logical ordering of a series of electrode channels on an EEG page. Ideally, an EEG montage is constructed such that it is easy to interpret by the reader, displays EEG information accurately, and limits the amount of electrical noise or artifact that obscures the tracing. In general, it is recommended that montages be set up such that channels are displayed with left hemispheric chains presented over right hemispheric chains and anterior electrodes over posterior electrodes, using consistent interelectrode distances.11 Bipolar Versus Referential Montages Imagine an EEG setup with five electrodes on the head, labeled A, B, C, D, and E. In a bipolar montage, the recording from the five electrodes is displayed in four channels: A–B, B–C, C–D, and D–E. In fact, the use of these “bipolar chains” is the most common strategy for displaying EEG signals. Alternatively, in a referential montage, each of the five electrodes is compared to a location on the body that is felt to be electrically quiet, such as the chin. For example, a referential montage using the chin for these five electrode positions would be displayed in five channels as: A-chin, B-chin, C-chin, D-chin, and E-chin. Electrodes that are not involved in the field of a discharge can also be used as a referential montage. For example, the Cz electrode can be used as a reference, where each electrode is compared to Cz (e.g., A–Cz, B–Cz, C–Cz, D–Cz, E–Cz). A summation of several electrodes can also be used, where each electrode is compared to the average electrical charge of all of the electrodes on the head (e.g., A–Avg, B–Avg, C–Avg, etc.). Most EEG screening is done in bipolar montages. Because the pairing of nearby electrodes in bipolar chains minimizes noise, bipolar montages tend to look “cleaner” and are easier to scan, although they do have inherent technical limitations. Localization of discharges and determination of whether a particular wave is artifact or of cerebral origin is often aided by reexamining an EEG page using a referential montage. Referential montages are more prone to electrical noise but have distinct advantages as well. In summary, a bipolar montage compares each electrode to a neighboring electrode, while a referential montage compares each electrode to a common electrode or summation of electrodes (e.g., average reference). 3Bipolar Montages The two types of bipolar montages used most often are anteroposterior (AP), in which electrode chains are set up from the front to the back of the head (Figure 1.2), and transverse, in which electrode chains are set up from the left to the right side of the head (Figure 1.3). This allows for scanning of the voltage differences in two different orthogonal planes across the scalp. The transverse bipolar montage also allows the reader to assess abnormalities at the ends of the electrode chains (e.g., O1/O2, Fp1/Fp2) more effectively than does the AP bipolar montage, given that, in an AP bipolar montage, end-of-chain electrodes are compared to only anterior (in the case of Fp1 and Fp2) or posterior electrodes (in the case of O1 and O2; Figure 1.3). Care should be used when creating montages to ensure that the interelectrode distance remains consistent in a bipolar montage whenever possible. Longer interelectrode distances create the effect of larger potential differences and apparent higher channel amplitudes (Figure 1.4), while shorter interelectrode distances create the effect of smaller potential differences and apparent lower channel amplitudes (Figure 1.5), even though the voltages generated by the underlying cortex are the same, creating a false asymmetry. If this is suspected, one should confirm the finding on a referential montage (Figure 1.5). Referential Montages When setting up a referential montage, how does one choose the ideal comparison point or reference electrode to compare to all the scalp positions? The ideal reference is one that “includes all of the electrical noise in the ‘electrode of interest’ but none of the electrocerebral activity.”12 The ideal comparison electrode would cancel out all of the noise from the scalp electrode of interest but would not inadvertently subtract out any of the electrical activity from the brain. Unfortunately, such an ideal reference electrode does not exist and compromises have to be made, but a reference position far enough from the electrode of interest to not contain much of its electrocerebral activity, but close enough to cancel out the common noise signal, is the goal. Possible reference electrode locations include the spinous process of C7 (CS2), the earlobes (A1 [left] and A2 [right]), the nose, or, as noted earlier, the chin or the vertex (Cz). Particularly in young children or those with sensory sensitivities, reference electrodes can be easily pulled off and are prone to myogenic artifact, though this may be less of a problem in ICU settings where patients are often comatose or sedated. As noted earlier, a more complex type of reference electrode can be created using a simple arithmetic average of the electrode set, referred to as an average reference electrode. With this scheme, a voltage that is the average of all of the scalp electrodes is attached to input 2 of the CMR. This strategy has its own inherent advantages and disadvantages. Average reference montages are most useful for localizing focal, low- to medium-voltage epileptiform discharges as these do not tend to affect the average very much. Broader discharges or those with high voltages tend to “contaminate” the average reference, leading to deflections in all channels to which it is compared and risking false localization.12 Special Situations/Limited Montages For some patients, it may be necessary to place a limited set of electrodes because some portions of the scalp may be inaccessible (due to surgical incisions or inability to turn the head). A custom montage may be created or a limited set of electrodes may be placed as a double-distance or even more limited electrode array. Limited montages also have the advantage of being simpler to apply, potentially permitting greater use of cEEG in resource-limited settings where EEG technologists may not be available or may be available in a limited capacity. The most common limited montage used in the ICU setting is the subhairline montage, in which stick-on electrodes are placed below the hairline by a bedside provider. These have been shown to have a high specificity for the detection of seizures and interictal epileptiform abnormalities; however, the sensitivity for seizure detection and epileptiform discharges is quite low. In a large study comparing full to limited-montage EEG, 170 adult ICU patients had an abbreviated montage of electrodes placed below the hairline and a full-montage 10-20 placement of electrodes as a reference.13 The 4-channel subhairline limited montage had a low sensitivity for the detection of both seizures (0.54, 95% confidence interval [CI] 0.29–0.77) and interictal epileptiform activity (0.60, 95% CI 0.46–0.74), although the specificity was high for seizures (1.00, 95% CI 0.97–1.00) and for interictal epileptiform activity (0.94, 95% CI 0.88–0.97). Similarly, a comparison of 70 patients using a 4-channel subhairline limited montage and a full-montage 10-20 placement of electrodes showed a sensitivity of 68% (95% CI 45–86%) and a specificity of 98% (95% CI 89–100%) for detection of seizures.14 Westover et al.15 hypothesized that discrepancies between limited and full montages may in part be due to inter-reader variability. It also appears that the configuration of the reduced montage impacts performance. A large study of a computational algorithm for the detection of ictal and other patterns showed a high sensitivity and specificity for a “hairline + vertex” montage and a “forehead + behind ear” montage in comparison to full electrode arrays.16 A study examining the performance of a headset-type continuous video EEG monitoring system from Nihon-Kohden (electrodes over the left and right frontal, temporal, central, and occipital regions) in comparison to a full-montage 10-20 placement of electrodes showed a sensitivity for detecting nonconvulsive status epilepticus of 0.706 (95% CI 0.440–0.897) with a specificity of 0.970 (95% CI 0.842–0.999).17 While 4potentially useful as a screening tool in limited resource settings, reduced electrode arrays do have limitations that must be considered when using these for the management of critically ill patients in the ICU. Principles of EEG Localization In bipolar montages, in which successive electrodes in a chain are sequentially paired, for example, A–B, B–C, the point of maximum negativity or positivity is found by identifying a phase reversal, or the point going through the chain where the waveform flips from down to up or up to down in successive channels (Figures 1.6A, 1.7A). When the wave flips from down to up and the tips in the phase-reversing channels are nearest to each other, this represents a point of maximum surface negativity. In instances where the waves flip from up to down and the waves appear to bulge away from each other, this represents a point of maximum surface positivity. It is important to remember that the concept of the phase reversal allows localization of not just epileptiform activity, but any type of wave, including slow waves, artifacts, and so on. In other words, the presence of a phase reversal does not provide evidence that a particular discharge is an epileptiform discharge; rather it identifies the point of maximum positivity or negativity on the scalp. The convention regarding the polarity or direction of deflection for EEG waves viewed on a referential montage is as follows: if the input 1 electrode is more negative than the input 2 electrode, the wave goes up, and if the input 1 electrode is more positive than the input 2 electrode, the wave goes down. This makes the approach to identifying the location of maximum voltage simple: the larger the wave, the greater the voltage. Assuming the ideal situation where the voltage at the referential electrode is more-or-less neutral, the largest up-going wave marks the location of the greatest negativity and, likewise, the largest down-going wave represents the location of maximum positivity. (Figures 1.6B and 1.7B).12 Visual Analysis of the EEG Many EEG tracings in the critical care setting are abnormal and reflect varying degrees of cerebral dysfunction. It is useful to be familiar with the appearance of the normal EEG in order to appreciate the degree to which any abnormal tracing deviates from normal. In Table 1.1, commonly used EEG descriptors are summarized.4,12,18 This section will briefly review the appearance of the normal pediatric EEG focusing on normal features and variants, especially those that could be confused for an abnormal pattern in the ICU. By 3 months of age, the normal EEG demonstrates an AP gradient during wakefulness. This term refers to the tendency of faster frequencies to be seen in the front of the head and slower frequencies in the back. The second feature of the gradient is that voltages tend to be higher in the posterior head regions and lower in the anterior regions. Thus, the AP gradient includes two separate gradients: decreasing frequency and increasing amplitude going from front to back. The simplest expression of this idea is that we often see low-voltage fast activity in the front of the head and slower, higher-voltage activity toward the back, in the form of the posterior rhythm or posterior dominant rhythm (PDR; Figures 1.8A and 1.9). One should note that the PDR has inherent asymmetry in voltage with lower voltage over the left as compared to the right hemisphere. If the amplitude of the PDR over the right hemisphere is more than three-fold greater than the amplitude of the PDR over the left hemisphere, it is considered abnormal. In contrast, if the amplitude of the PDR is greater than two-fold higher over the left hemisphere as compared to the right hemisphere, it is considered abnormal. This applies to patients who have a dominant left hemisphere. In patients with a dominant right hemisphere, the earlier amplitude criteria are reversed.12 The tracing should otherwise be symmetric, meaning that frequency and voltages are similar in homologous brain regions. Persistent asymmetry of normal background features is suggestive of a structural or functional abnormality in the hemisphere with the poorly developed or absent normal features. Reactivity should be present in all states, with a change in the EEG appreciated in response to external stimuli. Lack of these key characteristics is suggestive of encephalopathy and/or a structural or functional abnormality in the case of asymmetry.4 Typical ages of onset of normal background features are summarized in Table 1.2. A child should cycle through several states during a prolonged recording, including wakefulness, drowsiness or Stage 1 sleep (N1), Stage 2 sleep (N2), and deeper slow wave sleep (N3). Rapid eye movement (REM) sleep may also be seen (Figure 1.8B–H). Epileptiform discharges can be activated with transition from wakefulness into Stage 1 and Stage 2 sleep.4 Wakefulness Wakefulness is often associated with eye blink, muscle, and motion artifact in the EEG. The PDR is the primary identifiable rhythm seen during wakefulness. It appears with eye closure and disappears when the eyes are open and there is visual fixation (Figures 1.8A and 1.9). It first appears at 3 to 4 months of age with a frequency of 3 to 4 Hz and progressively increases to 10 Hz by 15 years of age. Table 1.3 summarizes the typical frequency of the PDR by age, as well as minimum normal values for age.19,21 5TABLE 1.1 Visual Analysis of Pediatric EEG
Descriptive Characteristic
Definition
Frequency
Rate at which a waveform repeats, measured in cps or Hz and often described in terms of specific frequency bands:
Delta: 0–<4 Hz
Theta: 4 to <8 Hz
Alpha: 8–13 Hz
Beta: >13 Hz–30 Hz
Gamma: >30 Hz
Voltage
Difference in amplitude between two points in a single channel derivation (e.g., Fp1–F7), calculated by multiplying the height of the wave on the page (mm) by the sensitivity (μV/mm) or using digital measurement tools
Duration
Time between waveforms or time from start to end of a single waveform
Polarity
Describes positivity or negativity of an electrical event on the scalp
Morphology
Shape of waveform (e.g., spike, sharp wave, sharply contoured, sinusoidal)
Location/Distribution
Description of which electrodes are involved in a waveform: focal (single or other highly restricted location), regional (multiple electrodes covering a portion of, but not the entirety of, a hemisphere), hemispheric (involving the entirety of the left or right hemisphere), generalized (entire cortex/scalp), or multifocal (three or more locations involving both hemispheres)
State
Awake, drowsy, or asleep
Symmetry
Similarity of waveforms in terms of amplitude, morphology, and frequency, comparing homologous regions of the left and right hemispheres
Rhythmicity
Measure of a wave’s regularity and predictability (e.g., rhythmic, semirhythmic)
Synchrony
Timing of waveforms in relation to one another, usually in reference to a comparison of the right and left hemisphere; waveforms may be synchronous (occurring simultaneously) or asynchronous (occurring 1.5–2 seconds apart)
Continuity
Describes waveforms that are uninterrupted by periods of voltage attenuation or suppression
Discontinuity
Describes waveforms that are interrupted by periods of voltage attenuation or suppression
Reactivity
A change in EEG pattern from an external stimulus; this may take a variety of forms, such as a transient change in the frequency or amplitude of the tracing
Stay updated, free articles. Join our Telegram channel
Full access? Get Clinical Tree
Basics of EEG Monitoring in Pediatric Critical Care
Coral M. Stredny and Mark H. Libenson
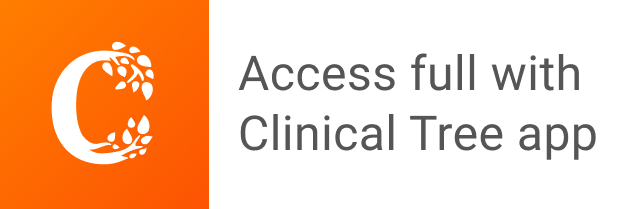