An important relationship between bilirubin and injury to the neonatal central nervous system has been recognized for many years. The first comprehensive description of the most overt form of bilirubin encephalopathy (i.e., kernicterus) was provided by Schmorl in 1903. The development of therapeutic measures, such as exchange transfusion, and of preventive measures, such as the use of anti-Rh immune globulin to prevent maternal sensitization, resulted in a marked decrease in this overt form of bilirubin encephalopathy. Subsequently implementation of predischarge universal screening coupled with the appropriate use of phototherapy and post–hospital discharge follow-up has been shown to be an extremely important strategy in the prevention of severe neonatal hyperbilirubinemia. The total impact of these strategies on hospital readmissions in the first 1 to 2 weeks of life remains to be defined.
In this chapter, normal bilirubin structure and metabolism, the pathophysiology of hyperbilirubinemia and bilirubin neurotoxicity, and the neuropathological and clinical aspects of bilirubin injury to the neonatal central nervous system are reviewed.
Normal Bilirubin Structure and Metabolism
Central to an understanding of the relation of bilirubin to neonatal brain injury is an awareness of the normal aspects of bilirubin structure, properties, and metabolism in the newborn. Thus, before the pathophysiology of bilirubin encephalopathies is discussed, certain highly relevant aspects of the chemical structure and solubility of bilirubin, as well as normal bilirubin metabolism, are briefly reviewed.
Bilirubin Structure and Properties
Bilirubin is a catabolic product of the porphyrin ring, derived from heme (see subsequent discussion). This compound can exist in plasma as bilirubin anion (monoanion or dianion form) ( Fig. 26.1A ) or as bilirubin acid (see Fig. 26.1B ). Bilirubin dianion binds actively to albumin. The dianion is not highly soluble in lipid or nonpolar solvents, but in view of the two polar carboxyl groups and oxidipyrryl (lactam) groups, it is not surprising that the anionic forms of bilirubin are relatively soluble in polar solvents. Although the anionic forms were considered previously to be the principal free bilirubin species in plasma, more recent work showed that at physiological pH the acceptance of two hydrogen ions results in bilirubin diacid (see Fig. 26.1B ). The diacid has a rigid folded structure, maintained by six internal hydrogen bonds involving all the polar groups, thereby rendering the molecule poorly soluble in aqueous solutions. However, the compound does passively diffuse across plasma membranes of cells (see later). When the concentration of free bilirubin diacid exceeds its limit of aqueous solubility (≈70 nM at physiological pH), the compound exists as soluble oligomers and metastable microaggregates. Moreover, at very high concentrations of the diacid, insoluble precipitates form that may result in major injury to membranes. At physiological pH , the species of free bilirubin are approximately 82% diacid, 16% monoanion, and 2% dianion. These chemical properties of bilirubin are important in understanding the neurotoxicity of bilirubin (see later discussion).

Bilirubin Metabolism
Normal bilirubin metabolism is considered best in terms of the following sequential events: (1) production, (2) transport, (3) hepatic uptake, (4) conjugation, (5) excretion, and (6) enterohepatic circulation ( Fig. 26.2 ).

Production
Bilirubin is the end product of the catabolism of heme, the major source of which is circulating hemoglobin (see Fig. 26.2 ). In the newborn infant, the normal destruction of circulating red blood cells in the reticuloendothelial system accounts for approximately 75% of the daily production of bilirubin. The conversion of the heme moiety to bilirubin requires the sequential action of two enzymes, heme oxygenase (to form biliverdin) and a reduced nicotinamide adenine dinucleotide phosphate (NADPH)–dependent biliverdin reductase (to form bilirubin). Approximately 25% of the daily production of bilirubin in the newborn is derived from sources other than senescent red blood cells. This other fraction has two major components: a nonerythropoietic component, resulting from turnover of nonhemoglobin sources of heme (e.g., cytochromes, catalase, peroxidase, and myoglobin), and an erythropoietic component, resulting from the destruction of products of ineffective erythropoiesis.
Transport
Bilirubin leaves the site of production in the reticuloendothelial system and is transported in plasma bound to albumin (see Fig. 26.2 ). Human albumin has a single, tight, high-affinity (or primary) binding site for bilirubin and one or more (probably two) weaker, lower-affinity binding sites. The capacity of serum albumin to bind bilirubin is known as the binding capacity , and the strength of the bilirubin-albumin bond is referred to as the binding affinity. The amount of free bilirubin (i.e., bilirubin not bound to albumin) is very low at physiological pH. These last three characteristics of a given serum-binding capacity, binding affinity, and amount of free bilirubin can be estimated by in vitro measurements and provide a measure, albeit only approximate, of the amount of bilirubin that may be available to cause neuronal injury (see later discussion).
Hepatic Uptake
Hepatocytes have a selective and highly efficient system for removing unconjugated bilirubin from plasma. This mechanism requires several different organic anion transport proteins. Variants of one of these, organic anion transporter 2 (OATP2), may be important in determining the elevated risk of severe hyperbilirubinemia in Asian infants. In the hepatocyte, the transported bilirubin is bound to ligandin, a cytosolic protein, that facilitates transfer to the endoplasmic reticulum, the site of bilirubin conjugation.
Conjugation
Conversion of bilirubin to excretable monoconjugates and diconjugates is carried out primarily by the microsomal enzyme uridine-diphosphate (UDP)–glucuronyl transferase (A1 isoform). The protein is encoded by the UGT1A1 gene. Variants of the UGT1A1 gene appear to be important in determining the elevated risk of severe hyperbilirubinemia in Asian infants. The disconjugate accounts for approximately 90% of total bilirubin glucuronide conjugates.
Excretion
The conjugated bilirubin is excreted into the bile. Because this event occurs across a concentration gradient, an energy-dependent active transport system is involved. The conjugated bilirubin is then transported to the small intestine, where it is primarily further degraded by intestinal bacteria and excreted in the stool.
Enterohepatic Circulation
Enterohepatic circulation also occurs. Intestinal beta-glucuronidase hydrolyzes the conjugated bilirubin, thus releasing free bilirubin, which is then reabsorbed and transported by the portal circulation to the liver (see Fig. 26.2 ).
Pathophysiology
Hyperbilirubinemia
Although the relationship between serum levels of unconjugated bilirubin and neurotoxicity is not simple (see subsequent sections), a general link can be discerned between neonatal hyperbilirubinemia and the risk of neural injury. The major causes of neonatal hyperbilirubinemia, including the universal ( physiological ) elevation of bilirubin in the newborn, are reviewed next.
Major Causes
In the newborn period, numerous disorders may lead to elevated concentrations of unconjugated bilirubin ( Table 26.1 ). These include the following: disorders with increased production , principally hemolytic disease, secondary to blood group incompatibility; intrinsic defects of red blood cells or hemoglobin; degradation of extravascular blood (i.e., hemorrhage); polycythemia; sepsis; disorders with disturbed gastrointestinal transit and therefore increased enterohepatic circulation; disorders of bilirubin conjugation , including inherited and acquired defects (e.g., prematurity); and hormonal disturbances (e.g., hypothyroidism). Central to the frequency of hyperbilirubinemia in the newborn is the development of hyperbilirubinemia that occurs normally and onto which these other causes are grafted. This physiological jaundice of the newborn may be better termed developmental hyperbilirubinemia because it is very difficult to define precisely when this neonatal process is physiological and when it is pathological.
Increased production |
Hemolytic disease |
|
Other |
|
Increased enterohepatic circulation |
|
Decreased conjugation |
|
Physiological Hyperbilirubinemia
Definition.
Physiological hyperbilirubinemia refers to the elevation of serum bilirubin values that occur in essentially every newborn infant in the first week of life. In classic earlier studies of formula-fed white and African American full-term infants, the serum bilirubin level rose gradually to a mean peak of approximately 6 mg/dL by 48 to 72 hours of life and then declined relatively rapidly to a slightly elevated level, approximately 3 mg/dL, at approximately 5 days of life. After the peak bilirubin level was reached, little change occurred for several days; then a second, gradual decline occurred, with normal levels reached by approximately 2 weeks. Thus, two phases could be identified in the human, and the mechanisms underlying these were studied in detail in the neonatal monkey, in which the biphasic course is also apparent. With the current increase in breast-feeding in the United States and elsewhere, peak values for bilirubin clearly are greater than in the earlier studies. Thus, in predominantly breast-fed white and African American term infants, the normal peak value is 8 to 9 mg/dL, and the decline is slower. In Asian newborns (predominantly breast-fed), the peak values, 10 to 14 mg/dL, are still higher than those in white and African American infants. In the premature infant, the peak serum bilirubin concentration occurs slightly later than in the full-term infant (i.e., approximately the fourth to fifth day) and is higher (i.e., mean: 10 to 12 mg/dL); normal levels may not be reached until 3 to 4 weeks of age. More importantly, the term physiological hyperbilirubinemia in the premature infant is misleading because such values are potentially dangerous (see later). Indeed, in infants of very low birth weight, phototherapy is recommended well before values reach 10 to 12 mg/dL (see later).
The late preterm infant (i.e., 35 to 37 weeks of gestation) presents a situation intermediate between the clearly premature infant and the full-term infant (38 to 42 weeks of gestation). Thus, several studies showed that such infants have a severalfold higher risk of significant hyperbilirubinemia in the first week of life. In a careful study through the first 7 days of life, near-term infants exhibited a later and higher peak value of bilirubin than did term infants. Moreover, the higher values declined more slowly in the near-term infants. Overall, 10% of the full-term infants had significant hyperbilirubinemia requiring phototherapy, versus 25% in the near-term group. These data highlight the importance of particularly close follow-up of near-term infants after hospital discharge (see later).
Mechanisms.
The mechanisms considered important in the genesis of physiological hyperbilirubinemia are shown in Table 26.2 . Although evidence has been mustered for all these factors, studies of the newborn monkey provided considerable insight into their relative importance. Thus, the first phase of hyperbilirubinemia (see previous section) has been shown to relate to the combined effects of (1) increased bilirubin load to the liver and (2) decreased bilirubin-conjugating capacity. The source of the increased bilirubin load to the liver includes both hemoglobin and nonhemoglobin sources as well as the enterohepatic circulation ; the latter is increased in the newborn because of deficient bacterial degradation of bilirubin and increased activity of intestinal beta-glucuronidase. The defective bilirubin conjugating capacity is related to a diminished activity of hepatic UDP-glucuronyl transferase, which undergoes a rapid developmental change from negligible levels at birth to adult levels after several days. Prematurity results in more severe neonatal hyperbilirubinemia, principally because of a delayed maturation of the hepatic UDP-glucuronyl transferase. More recent studies in human infants have refined the earlier observations. Thus the possibility of impaired transport of bilirubin into the hepatocyte because of genetic variants of organic anion transporters (see earlier) is likely important, perhaps especially in Asian infants. The diminished levels of ligandin in hepatic cytosol are likely less important. Genetic variants of the UGT1A1 gene as well as the long-recognized developmental deficiency of the glucuronyl transferase may also be important. Increase in the enterohepatic circulation is likely important in jaundice associated with breast milk feedings because breast milk contains beta-glucuronidase, which can degrade conjugated bilirubin in the small intestine. In addition, lipoprotein lipase activity in breast milk has been suggested to lead to the increased release of free fatty acids (anions) from triglycerides and thereby to a disturbance of hepatic uptake. Pregnanediol in breast milk may also inhibit bilirubin conjugation. Finally, the common delay in adequate caloric intake with breast-feeding is considered of most importance in jaundice associated with breast-feeding; the mechanism of the deleterious effect of caloric deprivation on bilirubin homeostasis remains unclear.
|
Important Determinants of Neuronal Injury by Bilirubin
The critical event in the genesis of brain injury caused by bilirubin is entrance of bilirubin into brain and exposure to neurons. The predominance of available data indicates that bilirubin per se and more specifically the bilirubin no longer bound to albumin, that is, unbound or “free” bilirubin , is the form that ultimately leads to neuronal injury (see later discussion).
Interrelationships of Bilirubin, Albumin, and Hydrogen Ion
To derive the important determinants of neuronal injury by bilirubin, it is necessary to recognize the critical reactions shown in Fig. 26.3 . As noted earlier, at physiological pH, the predominant species of unbound bilirubin in plasma is bilirubin acid. Consideration of these equilibria makes it clear that the potential means for increasing the exposure of neurons to bilirubin acid would include increasing the quantity of [bilirubin anion]-albumin (i.e., unconjugated bilirubin ) and especially thereby unbound or free bilirubin, disturbing the binding of bilirubin anion to albumin, decreasing the quantity of albumin that is free to bind bilirubin, and increasing the quantity of hydrogen ions (i.e., lowering pH) ( Table 26.3 ). Additional factors that interrelate closely with those just enumerated include the status of the blood-brain barrier and the susceptibility of target neurons to bilirubin injury; these factors are considered separately in later sections. In the following discussion of the determinants of bilirubin neurotoxicity, it becomes clear that the importance of each factor must vary with the clinical circumstances (see Table 26.3 ).

|
Concentration of Serum Unconjugated and Free Bilirubin
Unconjugated Bilirubin.
Although it is generally recognized that serum levels of unconjugated bilirubin must be elevated to cause neurotoxicity, the relationship between such elevations and brain injury is not simple. In the full-term infant with marked hyperbilirubinemia secondary to hemolytic disease , a clear correlation can be discerned between the occurrence of kernicterus and the maximal recorded level of serum bilirubin ( Table 26.4 ). In a review of 52 infants with hemolytic disease (33 Rh incompatibility, 19 ABO incompatibility) and comorbid factors, approximately 95% of whom developed kernicterus, the peak total serum bilirubin in both groups was approximately 32 mg/dL , with an approximate range of 18 to 51 mg/dL .
MAXIMUM BILIRUBIN CONCENTRATION (mg/dL) | TOTAL NO. OF CASES | NO. WITH KERNICTERUS |
---|---|---|
30–40 | 11 | 8 (73%) |
25–29 | 12 | 4 (33%) |
19–24 | 13 | 1 (8%) |
10–18 | 24 | 0 |
The neural risk of marked hyperbilirubinemia in full-term infants without hemolysis is less clear. Indeed, an earlier analysis of available studies by Newman and Maisels suggested that the risk for neurological sequelae is distinctly less for hyperbilirubinemic infants without hemolytic disease compared with the risk for infants with hemolytic disease. Subsequent observations supported this contention, including a study by Newman and associates of 140 treated infants (phototherapy n = 136) and exchange transfusion ( n = 5) with peak serum bilirubin levels largely between 25 and 29.9 mg/dL. There were no cases of kernicterus. However, multiple reports describe the occurrence of kernicterus, identified by neuropathological, neuroradiological, or clinical criteria, after apparent nonhemolytic hyperbilirubinemia. Furthermore, in a review of 35 selected infants with nonhemolytic, idiopathic hyperbilirubinemia and documented acute or chronic bilirubin encephalopathy, all infants had peak total serum bilirubin levels greater than 20 mg/dL ( Fig. 26.4 ). Indeed, more than 90% of the infants with kernicterus had peak levels higher than 25 mg/dL. Notably, however, fully 25% had peak levels lower than 30 mg/dL. Nevertheless, current observations suggest that hemolytic conditions are often overlooked in the absence of a detailed search for immune-mediated mechanisms by advanced techniques. It seems reasonable to conclude that hyperbilirubinemia, in most cases, is a necessary but usually not sufficient condition to explain kernicterus. Factors acting in concert with bilirubin, including duration of exposure to bilirubin or albumin binding of bilirubin, must be evaluated to seek a satisfactory explanation for the risk of developing kernicterus (see later). These issues are discussed in detail later (see the section on the relationship of neurological sequelae in term infants to degree of hyperbilirubinemia ).The relationships between neonatal bilirubin values and neurological outcome in premature infants are probably different from those in full-term infants. Indeed, kernicterus has been demonstrated repeatedly in the premature infant without marked hyperbilirubinemia (see later discussion). These infants have usually exhibited a variety of complicating illnesses (e.g., acidosis, hyperbilirubinemia, sepsis, asphyxia, hypothermia, intraventricular hemorrhage). In one often-cited, relatively large collection of premature infants with kernicterus ( n = 6), peak total serum bilirubin levels were in excess of 20 mg/dL (range, 22 to 26 mg/dL). However, in this report, the gestational age of the six infants ranged from 34 to 36 weeks. Studies of bilirubin-induced auditory disturbances in smaller premature infants (28 to 32 weeks of gestational age) document neurological dysfunction at much lower bilirubin levels. The critical issue of the premature infant is discussed later (see the section on clinical features ).

Free Bilirubin.
Because of the recognition that the fraction of bilirubin not bound to albumin is the critical component involved in bilirubin’s entry into the brain and in neurotoxicity (see later) and because of the demonstration of kernicterus in premature infants without markedly elevated levels of unconjugated bilirubin, intensive investigation has been directed toward measurement of the quantity of that fraction of unconjugated serum bilirubin, namely, unbound (i.e., free ) bilirubin. a
a References .
In general, premature infants with higher levels of free bilirubin exhibit kernicterus at postmortem examination more often than those with lower levels. In a recent study of 1100 infants of extremely low birth weight in whom total and unbound bilirubin levels were measured at 5 days, irrespective of the clinical status, an increasing level of unbound bilirubin was associated with higher rates of death, neurodevelopmental impairment, or hearing loss at 18- to 22-month follow-up.Disturbances of the brain stem auditory evoked response (BSAER) are observed at lower levels of free bilirubin in premature versus full-term infants (see later). Measuring the total bilirubin/albumin molar ratio (BAMR) was initially considered to be more clinically relevant and had been shown to be highly correlated with unbound bilirubin when the latter serum concentrations are less than but not greater than 0.6 µg/dL. However, in a recent randomized study in preterm infants of ≤32 weeks’ gestation with hyperbilirubinemia, no significant effect of the additional use of the bilirubin–albumin ratio compared with total serum bilirubin (TSB)–based treatment on the neurodevelopmental outcome at 18 to 24 months corrected age was observed. Thus it is clear that free bilirubin levels alone do not clearly distinguish premature infants who will develop kernicterus from those who will not. Moreover the use of phototherapy may in addition affect this relationship. Thus photoisomers, which account for up to 25% of the total bilirubin produced during phototherapy, may affect bilirubin–albumin binding, altering the level of unbound circulating bilirubin. The important point is that other determinants (e.g., concerning albumin-bilirubin binding, status of the blood-brain barrier, and neuronal susceptibility; see Table 26.3 ) are also often present in the sick premature infant and contribute to the likelihood of bilirubin injury (see subsequent sections). Experimental studies support a relationship between free or unbound bilirubin and neuronal injury.
Concentration of Serum Albumin
Consideration of the equilibria among albumin-bound bilirubin anion, albumin, hydrogen ion, and bilirubin acid (see Fig. 26.3 ) makes it apparent that the concentration of serum albumin is important in determining the neurotoxicity of bilirubin (see Table 26.3 ). At lower concentrations of serum albumin, the overall reaction favors the formation of unbound bilirubin anion and, ultimately, bilirubin acid. Indeed, in experimental systems, the toxic effects of bilirubin on enzymatic systems or on cultured cells of neural origin can be reversed by the addition of albumin. Moreover, evidence indicates that infants at the greatest risk for kernicterus in the absence of marked hyperbilirubinemia (i.e., sick premature infants) usually exhibit concentrations of serum albumin that are lower than those in healthy premature and full-term infants. Indeed, in one study of 27 premature infants with kernicterus, serum albumin levels were statistically significantly lower than in a comparable control group. However, the latter observation has not been entirely consistent, in keeping with the importance of such other factors as the bilirubin binding affinity and binding capacity of albumin (see following discussion).
Bilirubin Binding by Albumin
The capacity of serum albumin to bind bilirubin depends on such factors as the affinity of the albumin for bilirubin and the competition between bilirubin and other endogenous and exogenous anions for albumin binding sites. The clinical importance of the ability of albumin to bind bilirubin is emphasized by the demonstrations that premature infants who develop kernicterus without marked hyperbilirubinemia may have disturbances of the affinity or capacity, or both, of albumin to bind bilirubin. Similarly, a relationship between bilirubin–albumin binding and the subsequent cognitive outcome of premature infants who required neonatal intensive care further supports the clinical importance of this binding.
Affinity of Newborn Albumin for Bilirubin.
The affinity of albumin for bilirubin is less in the newborn than in the older infant. Adult levels of binding affinity are not reached until as late as 5 months of age. Moreover, binding affinity is lower in the premature infant than in the term infant and is lower in sick infants than in well infants. The explanation for the lower binding affinity of neonatal albumin is not entirely clear. The search for competing anions or for compositional differences in the protein as the unifying explanation has not been fruitful. The leading possibility is that a difference in conformation of the albumin is responsible. Moreover, it is likely that the conformational difference relates to the humoral environment of the neonatal albumin because adult serum albumin infused into newborns loses its superior binding affinity over 24 hours.
Endogenous Anions.
Endogenous anions that may compete with bilirubin for albumin binding sites include nonesterified fatty acids and other organic anions. a
a References .
Nonesterified fatty acids are anions at physiological pH and are present in high concentrations with hypothermia, hypoxemia, hypoglycemia, sepsis, starvation, the administration of heparinized blood (through heparin’s activation of triglyceride lipase), and intravenous alimentation with lipid. A recent report describes the uncoupling of unbound from total bilibulin as a result of unbound free fatty acids in premature infants treated with intralipids. Studies of asphyxiated infants with metabolic acidosis demonstrated impaired bilirubin binding to albumin that could not be attributed to the low pH per se. Rather, the data indicated the presence of organic anions in the plasma of asphyxiated, acidotic infants that compete with bilirubin for albumin binding sites. This finding may represent one of several mechanisms of potential importance in enhancing bilirubin neurotoxicity with asphyxia ( Table 26.5 ).
|
a Probable mechanisms are in parentheses; see text for references.
Exogenous Anions.
Many exogenous anions may compete with bilirubin for albumin binding sites. a
a References .
However, the largest proportion of such substances has been shown to displace bilirubin from albumin in vitro and in relatively high concentrations. Agents that appear to be particularly potent competitors for bilirubin binding sites include sulfonamides (especially sulfisoxazole), ibuprofen, and various penicillins (especially ceftriaxone). Indeed, ceftriaxone is a more potent bilirubin displacer than sulfisoxazole.Concentration of Hydrogen Ions: Acidosis
Results of experimental and clinical studies indicate that acidosis facilitates the neurotoxicity of bilirubin. Experimental data suggest that the principal deleterious effect of acidosis occurs at the level of cellular binding, at the uptake of bilirubin, or both. Thus enhanced binding and uptake of bilirubin in the presence of acidosis have been demonstrated with cells in tissue culture, liposomes, red blood cell membranes, rat brain slices, and rat brain in vivo. It is likely that the striking increase in brain bilirubin levels in neonatal rat brain observed during and shortly after anoxia also relates to acidosis. Moreover, the acidosis associated with asphyxia may be similarly important in enhancing the bilirubin neurotoxicity of that insult (see Table 26.5 ). The mechanism by which acidosis leads to increased neuronal binding, brain uptake of bilirubin, or both, presumably relates to the conversion of bilirubin anion to bilirubin acid (see Fig. 26.3 ) on acceptance of two hydrogen ions. Bilirubin anion binds to cellular surface membrane components, primarily phospholipids, but also gangliosides and sphingomyelin, and this binding is increased by decreased pH. This electrostatic interaction of the bilirubin anion and the cationic membrane lipid moieties is followed rapidly by the formation of bilirubin acid in the membrane, aggregation of bilirubin acid, irreversible binding to the membrane, and membrane injury, uptake, or both (see later discussion) ( Fig. 26.5 ). This effect of acidosis, when exerted at the level of the endothelial cells of the blood-brain barrier, perhaps could lead to increased transport of bilirubin across the barrier into the brain, in addition to the effect exerted at the level of the neuron (i.e., bilirubin acid binding, neuronal uptake, and neuronal death; see later sections concerning the blood-brain barrier and mechanisms of neurotoxicity ).

Blood-Brain Barrier
The entry of bilirubin into the central nervous system can potentially occur across the blood-brain or blood (choroid plexus)–cerebrospinal fluid barrier. It is likely, although not established, that transport across the blood-brain barrier is the more important of these two mechanisms. The blood-brain barrier is composed of the brain capillary endothelial cells with characteristic tight intercellular junctions. The choroid plexus does not contain such tight junctions. Export of bilirubin from the neurons and glia to the extracellular space and hence to the blood by capillary endothelial cells of the blood-brain barrier includes transport-mediated efflux across the blood-brain barrier. A similar process extrudes bilirubin from cerebrospinal fluid to blood through the cells of the choroid plexus. There are at least two types of transporters: ATP-binding cassette transporter B1 (ABCB1) P-glycoprotein, which is localized to the luminal (blood side) face of capillary endothelial cells of the blood-brain barrier, and ATP-binding cassette transporter C1 (ABCC1) multidrug resistance–associated protein 1 (MRP1), which is localized to the basolateral face of the choroid plexus epithelium of the blood–cerebrospinal fluid barrier. These transporters are upregulated with hyperbilirubinemia. However, disturbance in their function (e.g., genetic variants and possibly energy depletion) can render the brain in a given infant highly susceptible to the accumulation of bilirubin within neurons and glia, with resulting neurotoxicity. These transport mechanisms are summarized in Table 26.6 .
|
In this context, it is most reasonable to consider bilirubin entry into the brain across either an intact or a disrupted blood-brain barrier ( Table 26.7 ). The clinical circumstances largely determine the status of the blood-brain barrier (see Table 26.7 ).
|
a Question marks indicate experimental data that are suggestive but not yet conclusive.
Bilirubin Transport Across an Intact Blood-Brain Barrier.
The likelihood of the passage of bilirubin across an intact blood-brain barrier is suggested by the finding that free bilirubin binds to phospholipid (e.g., plasma membrane phospholipid of capillary endothelial cells). Such bilirubin-phospholipid complexes are very lipophilic ; thus they would be expected to move bilirubin across the blood-brain barrier readily ( Fig. 26.6 ). Therefore the aforementioned factors that lead to elevations of bilirubin no longer bound to albumin (i.e., free bilirubin) would be expected to enhance the movement of bilirubin across the blood-brain barrier. Studies in animals have demonstrated such movement across the intact blood-brain barrier, particularly in the younger as opposed to the more mature animal, and the occurrence of kernicterus in older children with apparently intact blood-brain barriers further supports this concept. As noted earlier, the predominant species of unbound bilirubin in plasma is the diacid, with the minority as the monoanion or dianion. These species readily diffuse across cell membranes. When free bilirubin is only moderately higher than its aqueous saturation (≈70 nM), soluble oligomers and metabolic microaggregates of the diacid form and bind to the outer leaflet of the plasma membrane. The result is a modest perturbation of membrane structure that may enhance the further uptake of bilirubin (see Fig. 26.5 ). These occurrences merge with the mechanism of bilirubin transport across a disrupted blood-brain barrier (see following section). Conditions such as acidosis that favor the formation of bilirubin acid (see Fig. 26.3 ) may be expected to provoke this series of events.

Studies of isolated brain capillary cells support this notion of toxicity of bilirubin to endothelia, with an associated effect on membrane transport.
A role for increased cerebral blood flow in the enhanced transport of bilirubin across an intact blood-brain barrier may be predicted if, as just discussed, bilirubin can be transported across an intact blood-brain barrier. Direct demonstration of an increase in bilirubin transport by an increase in cerebral blood flow was made in studies of neonatal piglets subjected to moderate hypercarbia ( Fig. 26.7 ). No increase in albumin uptake occurred; thus the blood-brain barrier remained intact under these conditions. The implications of this observation are considerable, because abrupt increases in cerebral blood flow are not uncommon in the premature infant, particularly the sick infant, with impaired cerebrovascular autoregulation (see Chapter 13 , Chapter 15 , Chapter 16 , Chapter 24 ).

Bilirubin Transport Across a Disrupted Blood-Brain Barrier.
The likelihood of the passage of bilirubin, even still bound to albumin, across a disrupted blood-brain barrier , caused by exposure to hyperosmolar materials , is suggested by the results of experimental studies, primarily in the rat (see Table 26.7 ). Thus, unilateral kernicterus was produced in the rat by the infusion of bilirubin-albumin after reversible opening of the blood-brain barrier was accomplished on the same side by the infusion of hypertonic arabinose. The infusion of hyperosmolar materials is a well-established means for disturbing endothelial tight junctions and thereby the blood-brain barrier. That it was indeed the bilirubin-albumin complex that entered the brain was shown by demonstrating an increase in brain concentration of albumin as well as bilirubin in the affected hemisphere. Subsequent studies confirmed the increase in brain bilirubin concentration under these conditions. An approximate similarity of the topography of the bilirubin deposition to that of kernicterus was reported in the initial work, but the critical question concerning whether the brain bilirubin deposition was followed by neuronal injury was not answered. However, subsequent studies did demonstrate alterations in electroencephalography and in brain energy metabolism parallel to the uptake of bilirubin ( Fig. 26.8 ). These data raise the possibility that sudden or sustained increases in serum osmolality (e.g., in the sick premature infant administered hypertonic glucose or sodium bicarbonate, in association with hypothalamic disturbance after asphyxia or intraventricular hemorrhage, or following an exchange transfusion) could lead to abrupt increases in brain bilirubin levels even in the absence of marked hyperbilirubinemia.

A second potential means of disrupting the blood-brain barrier in the newborn is hypercarbia (see Table 26.7 ). Thus experimental data suggest that hypercarbia can disturb the blood-brain barrier and induce kernicterus in puppies. A careful study of the rat demonstrated that marked hypercarbia (carbon dioxide pressure [P co 2 ], 100 mm Hg), independent of acidosis, caused a more than twofold increase in brain bilirubin concentration as well as in the brain albumin level. The mechanism of the effect of hypercarbia could involve the impact of maximal vasodilation and an abrupt increase in cerebral blood flow on the integrity of the blood-brain barrier. These data raise the possibility that hypercarbia in the sick premature infant could contribute to the transport of bilirubin into the brain of the infant without marked hyperbilirubinemia.
Asphyxia may lead to disruption of the blood-brain barrier, at least in mature animals (see Table 26.7 ). Moreover, kernicterus has been produced readily in the asphyxiated but not in the nonasphyxiated monkey or rabbit. In addition, in the Gunn rat, it leads to hearing impairment at levels of bilirubin otherwise insufficient to lead to injury. It is tempting to suggest that the association of kernicterus and asphyxia in the human infant could stem, at least in part, on a disturbance in the blood-brain barrier. However, the only direct measurement of albumin transport across the blood-brain barrier in an asphyxiated perinatal animal involved the fetal lamb, and no significant increase in transport could be demonstrated. The possibility that asphyxia, by way of the associated acidosis, could lead to the enhanced formation of bilirubin acid at the endothelial cell surface, injury to the blood-brain barrier, and the transport of bilirubin acid (see previous section) remains to be determined. Moreover, the marked hypercarbia associated with asphyxia could lead to increased brain uptake of bilirubin through both an intact blood-brain barrier, as described earlier, and a disrupted blood-brain barrier, as just described. Finally, the ATP-dependent transporters on brain endothelial cells (see Table 26.6 ) perhaps could be impaired by the energy depletion of asphyxia. This issue requires further study, but it may add to the mechanisms of potentiation of bilirubin neurotoxicity with asphyxia (see Table 26.5 ).
Intracranial infection , particularly meningitis, is associated with vasculitis and a clearly compromised blood-brain barrier (see Table 26.7 ). A possible role for this mechanism in the genesis of kernicterus is suggested by clinical data. Thus, in two affiliated neonatal facilities, four infants with kernicterus were observed at postmortem examination over 5 years; of these four babies, all exhibited sepsis and two had meningitis. Whether the effect of sepsis-meningitis was at the level of the blood-brain barrier, enhanced neuronal susceptibility to bilirubin injury because of concomitant neuronal injury (see later discussion), or a combination of these factors requires further study. In a more recent report, the presence of sepsis (odds ratio [OR] = 20.6) greatly increased the risk of acute bilirubin encephalopathy.
Finally, the possibility that the blood-brain barrier can be disrupted transiently by abrupt increases in blood pressure, especially in the infant with impaired autoregulation, or by increased venous pressure , with an associated increase in transport of bilirubin into brain, is suggested by several lines of evidence (see Table 26.7 ). First, as noted earlier, circumstances that cause an increase in cerebral blood flow, such as hypercarbia, are associated with the increased transport of bilirubin into brain. An abrupt increase in blood pressure in the sick infant with a pressure-passive cerebral circulation would be expected to cause an abrupt increase in cerebral blood flow (see Chapters 13 and 24 ). Seizure, which causes an abrupt increase in blood pressure and cerebral blood flow in the newborn (see Chapter 12 ), may also lead to transient disruption of the blood-brain barrier. Such a disruption was documented shortly after a seizure in the human adult and was delineated carefully in animal models. Abrupt increases in venous pressure are common in sick premature infants, particularly in relation to respiratory disturbances (see Chapter 24 ). More data are needed concerning the possibility that transient disruptions in the blood-brain barrier are an important means for the entry of bilirubin into the neonatal brain.
Neuronal Susceptibility
The distinctive regional topography of brain injury with kernicterus (see subsequent discussion) and the predilection for involvement of neurons indicate a selective susceptibility of specific neurons to bilirubin injury. The mechanisms accounting for this selective susceptibility are unclear and cannot be explained solely by bilirubin transport across the blood-brain barrier, particularly the disrupted barrier, or with patterns of highest regional cerebral blood flow or metabolic rate.
The predilection of bilirubin injury for neurons rather than glia (see later discussion of neuropathology ) was reproduced in cultured cells. This predilection may relate to aspects of neuronal surface membranes (e.g., abundance of gangliosides) that lead more readily to binding of bilirubin and to the initial steps leading to membrane injury (see later discussion of mechanisms of neurotoxicity ). This speculation is only one of many possible explanations, however.
Perhaps of particular clinical importance, available data suggest that concomitant injury to neurons enhances the likelihood of bilirubin encephalopathy. The potentiating role of asphyxia may be a good example of this effect (see Table 26.5 ). Thus, in this circumstance, concomitant injury to neurons may render the cell more susceptible to uptake of and injury by bilirubin, and, as will be discussed regarding mechanisms of neurotoxicity, exposure of neurons to bilirubin may enhance the susceptibility to hypoxic-ischemic excitotoxic neuronal injury. As noted earlier, these factors related to neuronal susceptibility are among several other factors that may underlie the increased likelihood of bilirubin encephalopathy with asphyxia (see Table 26.5 ). Nevertheless, it also must be considered that other insults to neurons by such factors as hypoglycemia, metabolic aberrations, intracranial hemorrhage, infection, trauma, or exposure to toxic agents may have critical additive effects with bilirubin by producing concomitant injury to neurons. The role of such factors in enhancing neuronal susceptibility to injury could be very important and requires further study. The demonstrations that bilirubin toxicity to cultured cells is enhanced by otherwise nontoxic concentrations of tumor necrosis factor-alpha (TNF-α) and endotoxin (lipopolysaccharide) suggest a mechanism whereby sepsis-meningitis could enhance neuronal susceptibility to bilirubin injury. The role of inflammatory mechanisms in bilirubin-induced excitotoxicity is also relevant to this issue (see later). Finally, the observation that immature rat brain is rich in heme oxygenase, which could lead ultimately to the formation of bilirubin from local heme sources, raises the possibility that certain neurons could be exposed to particularly high local concentrations of bilirubin. Further information concerning the factors that could activate heme oxygenase, increase availability of its substrate, or both would be of interest concerning neuronal susceptibility to bilirubin injury.
Nonneuronal cells, including especially astrocytes and microglia, also show sensitivity to unconjugated bilirubin, and their responses may play a role in modulating the toxicity of bilirubin to neurons. The interactions of these cells are described later (see potential sequence for bilirubin neurotoxicity ). Bilirubin toxicity to other cellular structures including unmyelinated axons and differentiating oligodendrocytes is suggested by several experimental studies. Relevance to the human neuropathology (see later) remains to be clarified.
Mechanisms of Bilirubin Neurotoxicity
Spectrum of Effects of Bilirubin on Cellular Functions
The mechanism of injury of neurons by bilirubin is not entirely resolved, but recent work has provided important insights (see later). Previous work indicated that bilirubin exerts a deleterious effect on a wide variety of cellular events. Various in vitro and in vivo studies, primarily with animals, including Gunn rats, and more recently a genetically engineered mouse model created by introducing a premature stop codon in the Ugt1a1 gene and resulting in an inactive glucuronyl transferase, have demonstrated disturbances in glucose utilization, oxidative phosphorylation, glycogen synthesis, citric acid cycle function, cyclic adenosine monophosphate synthesis, amino acid and protein metabolism, DNA synthesis, lipid metabolism, myelination, synthesis and transport of neurotransmitters, ion transport, synaptic transmission, excitatory amino acid homeostasis, cytosolic calcium levels, nitric oxide release, free radical homeostasis including glutathione content, inflammatory homeostasis, and apoptotic/survival balance ( Table 26.8 ). a
a References .
A recent case report of a 32-week 1600-g premature infant with total serum bilirubin levels of 13.1, 28.8, and 21.4 mg/dL on the first, second, and fourth postnatal days and who died on the fourth day may add further insight into the cellular and molecular changes contributing to bilirubin-induced neuropathology. Compared with age-matched controls, increased blood vessel density with ill-defined luminal structures was observed in mesencephalon, pons, medulla corpus striatum, and hippocampus. The last two regions exhibited increased expression of vascular endothelial growth factor (VEGF) and vascular endothelial growth factor receptor-2 (VEGFR-2) as well as albumin extravasation into brain parenchyma.Impairment of
|
a Based on numerous studies performed in vivo and in vitro (cultured cells, tissue extracts); see text for references.
A particular importance for disturbance of mitochondrial function and thereby energy metabolism has been suggested by several studies using biochemical measurements and magnetic resonance (MR) spectroscopy to analyze energy metabolites, including high-energy phosphate levels. Thus a rapid decline in high-energy phosphate levels was observed in brain 15 minutes after the entrance of bilirubin by disruption of the blood-brain barrier ( Table 26.9 ). The findings were indicative of a disturbance in oxidative phosphorylation, with the depletion of glucose and glycogen and accumulation of lactate indicative of an increase in glycolytic rates in an attempt to restore energy potential. Moreover, similar experiments, also using MR spectroscopy, documented in vivo the disturbance in high-energy phosphate levels (see Fig. 26.8 ). The importance of hypoxia in potentiating the effect of bilirubin on energy metabolism was also shown by MR spectroscopy in a similar model ( Fig. 26.9 ). In addition, brief exposure of immature primary cortical neurons to bilirubin rapidly and selectively inhibited the mitochondrial respiratory chain at the level of the cytochrome c oxidase complex. This effect resulted in an impairment in oxygen consumption, inner mitochondrial membrane energy failure, and apoptosis. In addition, there was an increase in free radicals—that is, oxidized glutathione and superoxide anion. The antioxidant glycoursodeoxycholic acid blocked the inhibitory effects on cytochrome c activity with prevention of oxidative stress, metabolic alterations, and cellular death.
METABOLITE | CONTROL | BILIRUBIN |
---|---|---|
Phosphocreatine | 4.93 | 2.04 |
Adenosine triphosphate | 2.83 | 1.72 |
Glycogen | 2.82 | 1.20 |
Glucose | 2.95 | 1.02 |
Lactate | 2.27 | 10.55 |
a Values are mmol/kg brain tissue; all differences are significant at the P < .05 level.

Involvement of excitotoxic mechanisms in bilirubin-induced injury emanated initially from the demonstrations in the Gunn rat that (1) bilirubin-induced neuronal injury could be blocked by administration of the N -methyl- d -aspartate (NMDA) receptor antagonist MK-801 and (2) excitotoxic neuronal injury induced by injection of NMDA into striatum is accentuated by bilirubin entry into the region. These observations suggested that excitotoxic mechanisms could contribute to the neuronal injury caused by bilirubin. Recent experimental evidence suggests that as in hypoxia-induced excitotoxicity, the final common pathway to cell death involves the generation of reactive oxygen and nitrogen species (see Chapter 13 ). Thus, using rat cortical neurons, the role of nitric oxide (NO) and NMDA glutamate receptors in bilirubin neurotoxicity was evaluated. Bilirubin increased the expression of neuronal nitric oxide synthase (nNOS), as well as the production of nitrites and cyclic guanosine monophosphate (GMP), which was accompanied by protein oxidation and cell demise, whereas the major cellular antioxidant defense system, provided by glutathione, was impaired. Moreover, bilirubin-induced neuronal oxidative injury was prevented by NO synthesis inhibition as well as by blockade of the NMDA receptor channel with MK-801. These findings point to NO as a key mediator of bilirubin-induced neuronal oxidative injury and suggests that the upregulation of nNOS and neurotoxicity occur through the NMDA receptor. The sequence of events then could be similar to that described in hypoxia-ischemia (see Chapter 13 ) and in hypoglycemia (see Chapter 25 ), whereby a disturbance in high-energy phosphate levels leads to impairment of energy-dependent glutamate uptake mechanisms in both nerve terminals and astrocytes, a resulting accumulation of extracellular glutamate, and neuronal death initiated by activation of glutamate receptors, particularly the NMDA receptor. The demonstration of an impairment of glutamate uptake mechanisms by synaptic vesicles treated with bilirubin suggested that bilirubin itself could cause or accentuate the increase in extracellular glutamate. These considerations provided an additional reason for a link between hypoxic-ischemic insults and bilirubin neuronal injury (see Table 26.5 ). Moreover, the finding that the combination of lipopolysaccharide and bilirubin exacerbated excitotoxic neuronal injury induced by bilirubin suggests a mechanism whereby sepsis could increase the risk of kernicterus.
The involvement of inflammatory mechanisms in bilirubin-induced injury is based primarily on studies of experimental models. The pivotal roles of microglia and astrocytes are apparent. Thus microglia exposed to unconjugated bilirubin become activated and secrete multiple inflammatory cytokines, potentially injurious to neurons. A neuroprotective effect of the antimicroglial agent minocycline in Gunn rats supports a deleterious role of such an inflammatory mechanism. An important role for astrocytes in inducing the inflammatory response is suggested by the demonstration that astrocytes exposed to bilirubin release multiple inflammatory cytokines. Immature astrocytes release more TNF-α than more mature cells. These glia-mediated inflammatory mechanisms would be expected to contribute importantly to bilirubin-induced neurotoxicity both by accentuating excitotoxicity and generating free radicals (see Chapter 14 ).
Potential Sequence for Bilirubin Neurotoxicity
The observations of bilirubin-induced energy depletion, excitotoxicity, and free radical attack just reviewed should be viewed in the context of a broader cellular and molecular scheme ( Fig. 26.10 ). Since the early 2000s, studies based on concentrations of free bilirubin more relevant to the clinical setting suggest that the principal subcellular compartments affected are the plasma membrane and the mitochondrion and that the major cell types involved are the neuron and the astrocyte (see earlier).

Plasma Membrane and Excitotoxicity.
Although bilirubin dianion and its rapidly formed diacid can bind to phospholipids and diffuse passively across the plasma membrane, disturbance of the plasma membrane can also occur. The results are accentuation of bilirubin uptake and disturbance of certain plasma membrane functions (e.g., glutamate transport). Impairment of glutamate transport in neurons and especially astrocytes causes an increase in extracellular glutamate (see earlier). The result is excessive activation of neuronal NMDA receptors with an increase in cytosolic calcium, the generation of free radicals, and neuronal necrosis, apoptosis, or both as described for hypoxia-ischemia in Chapter 13 . Prevention of bilirubin-induced injury by specific inhibitors of the NMDA receptor (e.g., MK-801) supports this formulation.
Astrocytes also sustain injury from unconjugated bilirubin, although their vulnerability to cell death is less than that for neurons. Bilirubin induces a rapid increase in extracellular glutamate content, predominantly via immature astrocytes, that appears to result from several factors. First, impairment of glutamate transport is greater in astrocytes than in neurons; thus the accumulation of extracellular glutamate attributable to astrocytic dysfunction is greater than that for neurons (see Fig. 26.10 ). Second, astrocytes exposed to bilirubin release cytokines such as TNF-α and interleukin-1beta, which also impair glutamate transport; therefore the action of these cytokines may add to the accumulation of extracellular glutamate (see Fig. 26.10 ). Immature astrocytes also release more TNF as compared with the more differentiated cells. In addition, bilirubin-induced activation of NF-kappa B has an age-dependent profile, an effect that can be related to the greater amount of cytokine secretion by these cells. Third, because high-affinity sodium-dependent glutamate transport is impaired secondary to energy depletion and the resulting disturbance of ionic gradients (see Chapter 13 ), the energy depletion associated with bilirubin mitochondrial toxicity (see later) adds to the transport failure (or even reversal of transport) and accumulation of extracellular glutamate.
Importance of the Mitochondrion.
In addition to the plasma membrane and excitotoxic effects, bilirubin exposure in clinically relevant concentrations has important effects on mitochondria (see Fig. 26.10 ). What results is not only diminished energy production but also permeabilization of mitochondrial membranes and release of cytochrome c . The latter then leads to apoptotic neuronal death by binding to apoptosis protease activating factor-1, activation of caspases, and execution of the cell death program (see earlier).
Final Common Pathway to Cell Death.
The final common pathway for both the plasma membrane effects with excitotoxicity and the mitochondrial disturbances includes an increase in cytosolic calcium and the generation of free radicals (see Fig. 26.10 ) (see Chapter 13 ). The result is apoptotic cell death, necrotic cell death, or both. Both neurons and astrocytes can be affected, although neurons are more vulnerable. The intensity and duration of the stimulus determine whether neuronal death will be apoptotic or necrotic (see Chapter 13 ). High bilirubin concentrations, longer exposures, or both result in necrosis and lower concentrations or shorter exposures result in apoptosis. A particular although not exclusive importance for apoptotic cell death with bilirubin toxicity was suggested by the observation that inhibition of the proapoptotic enzyme p38 MAP kinase by minocycline prevented cerebellar neuronal cell death with bilirubin exposure. (This effect was likely independent of the antimicroglial effects of minocycline, as discussed in Chapter 13 .) Moreover, minocycline administered to the newborn Gunn rat prevented the marked apoptotic cell death that resulted in cerebellar hypoplasia in that animal. Combination of antiapoptotic (caspase inhibitor) and antiexcitoxic (MK-801) agents may exhibit synergy in neuronal protection from bilirubin toxicity.
Implications for Therapy
The sequence outlined in Fig. 26.10 and the supporting experimental data suggest several potential sites for therapeutic intervention ( Table 26.10 ). Thus blockade of NMDA receptors has been shown to be highly effective, depending on the experimental model. MK-801 is the most effective NMDA inhibitor but unfortunately is not clinically safe (see Chapters 13 and 20 ). However, memantine, magnesium, or dextromethorphan would be potentially more useful. Scavenging of free radicals with such agents as vitamin E or related compounds or enhancing antioxidant defenses with antioxidant enzyme mimetics may be of potential value. Antiapoptotic interventions may also be beneficial (see Chapter 20 ), and the beneficial effects of minocycline in two rat models are relevant in this context. Combinations of interventions may have synergistic effects, as noted earlier for antiapoptotic (caspase inhibitor) and antiexcitoxic (MK-801) agents. At any rate, insights into the mechanisms of bilirubin neurotoxicity raise important new possibilities for prevention of this injury. Nonetheless the most important therapeutic intervention is the rapid lowering of the bilirubin level with either phototherapy and/or exchange transfusion (see later).
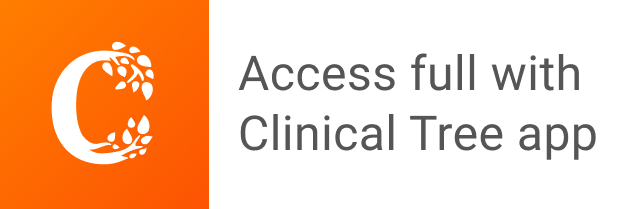