The human nervous system is the organ of consciousness, cognition, ethics, and behavior; as such, it is the most intricate structure known to exist. More than one-third of the 23,000 genes encoded in the human genome are expressed in the nervous system. Each mature brain is composed of 100 billion neurons, several million miles of axons and dendrites, and >1015 synapses. Neurons exist within a dense parenchyma of multifunctional glial cells that synthesize myelin, preserve homeostasis, and regulate immune responses. Measured against this background of complexity, the achievements of molecular neuroscience have been extraordinary. This chapter reviews selected themes in neuroscience that provide a context for understanding fundamental mechanisms underlying neurologic disorders.
The landscape of neurology has been transformed by modern molecular genetics. Several hundred neurologic and psychiatric disorders can now be diagnosed through genetic testing (http://www-ncbi-nlm-nih-gov/sites/GeneTests/?db=GeneTests). The vast majority of these represent highly penetrant mutations that cause rare neurologic disorders; alternatively, they represent rare monogenic causes of common phenotypes. Examples of the latter include mutations of the amyloid precursor protein in familial Alzheimer’s disease, the microtubule-associated protein tau (MAPT) in frontotemporal dementia, and α-synuclein in Parkinson’s disease. These discoveries have been profoundly important because the mutated gene in the familial disorder often encodes a protein that is also pathogenetically involved (although not mutated) in the typical, sporadic form. The common mechanism involves disordered processing and, ultimately, aggregation of the protein leading to cell death (see “Protein Aggregation and Neurodegeneration,” below).
There is optimism that complex genetic disorders, caused by combinations of both genetic and environmental factors, have now become tractable problems. Genome-wide association studies (GWAS) have been carried out in many complex neurologic disorders, with many hundreds of variants identified, nearly all of which confer only a small increment in disease risk (1.15- to 1.5-fold). GWAS studies are rooted in the “common disease, common variant” hypothesis, as they examine potential risk alleles that are relatively frequent (e.g. >5%) in the general population. More than 1500 GWAS studies have been carried out, with notable successes such as the identification of 110 risk alleles for multiple sclerosis (Chap. 45). Furthermore, using bioinformatics tools, risk variants can be aligned in functional biologic pathways to identify novel pathogenic mechanisms as well as to reveal heterogeneity (e.g., different pathways in different individuals). Despite these successes, many experienced geneticists question the real value of common disease-associated variants, particularly whether they are actually causative or merely mark the approximate locations of more important—truly causative—rare mutations.
This debate has set the stage for the next revolution in human genetics, made possible by the development of increasingly efficient and cost-effective high-throughput sequencing methodologies. It is already possible to sequence an entire human genome in approximately an hour, at a cost of only $1300 for the entire coding sequence (“whole-exome”) or $3000 for the entire genome; it is certain that these costs will continue to decline. This makes it feasible to look for disease-causing sequence variations in individual patients with the possibility of identifying rare variants that cause disease. The utility of this approach was demonstrated by whole-genome sequencing in a patient with Charcot-Marie-Tooth (CMT) neuropathy, in which compound heterozygous mutations were identified in the SH3TC2 gene, which then were shown to co-segregate with the disease in other members of the family.
It is increasingly recognized that not all genetic diseases or predispositions are caused by simple changes in the linear nucleotide sequence of genes. Disease-causative mutations also occur commonly in noncoding sequences of DNA. For example, large intronic GGGGCC repeat expansions in a gene of unknown function C9orf 72 (chromosome 9 open reading frame 72) were recently identified as a common cause of both frontotemporal dementia and amyotrophic lateral sclerosis (ALS). This mutation is the most common cause of both familial and sporadic ALS identified thus far. It was shown to be associated with TDP-43 (tar DNA binding protein-43) inclusions in both hippocampal and cerebral neurons. Interestingly, despite the absence of a start codon, the three alternate dipeptide sequences consisting of two amino acids are translated and found in postmortem brain tissue of affected patients. Three potential pathogenic mechanisms have been proposed, including (1) haploinsufficiency, (2) repeat RNA-mediated toxicity, and (3) dipeptide protein toxicity. The possibility of RNA toxicity is supported by the finding of intranuclear RNA foci containing C9orf 72 hexanucleotide repeats and specific RNA-binding proteins. The C9orf 72 mRNA forms quadruplexes of DNA and RNA, which then can halt transcription and also bind transcription factors. Mutations in both TARDP (transactive region DNA binding protein) and FUS (fused in sarcoma) also encode DNA/RNA-processing polypeptides and are a cause of familial and sporadic ALS.
As the complex architecture of the human genome becomes better defined, many disorders that result from alterations in copy numbers of genes (“gene-dosage” effects) resulting from unequal crossing-over are also likely to be identified. As much as 5–10% of the human genome consists of nonhomologous duplications and deletions, and these appear to occur with a much higher mutational rate than is the case for single base pair mutations. The first copy-number disorders to be recognized were Charcot-Marie-Tooth disease type 1A (CMT1A), caused by a duplication in the gene encoding the myelin protein PMP22, and the reciprocal deletion of the gene causing hereditary liability to pressure palsies (Chap. 53). Gene-dosage effects are causative in some cases of Parkinson’s disease (α-synuclein), Alzheimer’s disease (amyloid precursor protein), spinal muscular atrophy (survival motor neuron 2), the dysmyelinating disorder Pelizaeus-Merzbacher syndrome (proteolipid protein 1), late-onset leukodystrophy (lamin B1), and a variety of developmental neurologic disorders. It is likely that copy-number variations contribute substantially to normal human genomic variation for numerous genes involved in neurologic function, regulation of cell growth, and regulation of metabolism. It is also already clear that gene-dosage effects will influence many behavioral phenotypes, learning disorders, and autism spectrum disorders. Deletions at ch30q and ch15q have been associated with schizophrenia, and deletions at 15q and 16p with autism. Interestingly, the 16p deletion is also associated with epilepsy. Duplications of the X-linked MeCP2 gene cause autism in males and psychiatric disorders with anxiety in females, whereas point mutations in this gene produce the neurodevelopmental disorder Rett’s syndrome. The understanding of the role of copy number variation in human disease is still in its infancy.
The role of splicing variation as a contributor to neurologic disease is another area of active investigation. Alternative splicing refers to the inclusion of different combinations of exons in mature mRNA, resulting in the potential for many different protein products encoded by a single gene. Alternative splicing represents a powerful mechanism for generation of complexity and variation, and this mechanism appears to be highly prevalent in the nervous system, affecting key processes such as neurotransmitter receptors and ion channels. Numerous diseases are already known to result from abnormalities in alternative splicing. Increased inclusion of exon 10–containing transcripts of MAPT can cause frontotemporal dementia. Aberrant splicing also contributes to the pathogenesis of Duchenne’s, myotonic, and facioscapulohumeral muscular dystrophies; ataxia telangiectasia; neurofibromatosis; some inherited ataxias; and fragile X syndrome, among other disorders. It is also likely that subtle variations of splicing will influence many genetically complex disorders. A splicing variant of the interleukin 7 receptor α chain, resulting in production of more soluble and less membrane-bound receptor, is associated with susceptibility to multiple sclerosis (MS) in multiple different populations.
Epigenetics refers to the mechanisms by which levels of gene expression can be exquisitely modulated, not by variations in the primary genetic sequence of DNA but rather by postgenomic alterations in DNA and chromatin structure, which influence how, when, and where genes are expressed. DNA methylation and methylation and acetylation of histone proteins that interact with nuclear DNA to form chromatin are key mediators of these events. Epigenetic processes appear to be dynamically active even in postmitotic neurons. Imprinting refers to an epigenetic feature, present for a subset of genes, in which the predominant expression of one allele is determined by its parent of origin. The distinctive neurodevelopmental disorders Prader-Willi syndrome (mild mental retardation and endocrine abnormalities) and Angelman’s syndrome (cortical atrophy, cerebellar dysmyelination, Purkinje cell loss) are classic examples of imprinting disorders whose distinctive features are determined by whether the paternal or maternal copy of the chromosome of the critical genetic region 15q11-13 is responsible. In a study of discordant monozygotic twins for MS in which the entire DNA sequence, transcriptome (e.g., mRNA levels), and methylome were assessed genome-wide, tantalizing allelic differences in the use of the paternal, compared to maternal, copy for a group of genes were identified. Preferential allelic expression, whether due to imprinting, resistance to X-inactivation, or other mechanisms, is likely to play a major role in determining complex behaviors and susceptibility to many neurologic and psychiatric disorders.
Another advance is the development of transgenic mouse models of neurologic diseases, which has been particularly fruitful in producing models relevant to Alzheimer’s disease, Parkinson’s disease, Huntington’s disease, and ALS. These models are useful in both studying disease pathogenesis and developing and testing new therapies. New transgenic mouse models with conditional expression have fostered investigations in which late gene expression avoids developmental compensation or in which the reversibility of a disease phenotype can be examined by turning a gene off after the disease phenotype has manifested. One can also examine the effects of gene expression in specific subsets of neurons, such as entorhinal cortex, or selectively in neurons, astrocytes, or microglia. Models in both Caenorhabditis elegans and Drosophila have also been extremely useful, particularly in studying genetic modifiers and therapeutic interventions.
The resting potential of neurons and the action potentials responsible for impulse conduction are generated by ion currents and ion channels. Most ion channels are gated, meaning that they can transition between conformations that are open or closed to ion conductance. Individual ion channels are distinguished by the specific ions they conduct; by their kinetics; and by whether they directly sense voltage, are linked to receptors for neurotransmitters or other ligands such as neurotrophins, or are activated by second messengers. The diverse characteristics of different ion channels provide a means by which neuronal excitability can be exquisitely modulated at both the cellular and the subcellular levels. Disorders of ion channels—channelopathies—are responsible for a growing list of human neurologic diseases (Table 30-1). Most are caused by mutations in ion channel genes or by autoantibodies against ion channel proteins. One example is epilepsy, a syndrome of diverse causes characterized by repetitive, synchronous firing of neuronal action potentials. Action potentials are normally generated by the opening of sodium channels and the inward movement of sodium ions down the intracellular concentration gradient. Depolarization of the neuronal membrane opens potassium channels, resulting in outward movement of potassium ions, repolarization, closure of the sodium channel, and hyperpolarization. Sodium or potassium channel subunit genes have long been considered candidate disease genes in inherited epilepsy syndromes, and recently such mutations have been identified. These mutations appear to alter the normal gating function of these channels, increasing the inherent excitability of neuronal membranes in regions where the abnormal channels are expressed.
CATEGORY | DISORDER | CHANNE 1 TYPE | MUTATED GENE | CHAP. REF. |
---|---|---|---|---|
Genetic | ||||
Ataxias | Episodic ataxia-1 Episodic ataxia-2 Spinocerebellar ataxia-6 | K Ca Ca | KCNA1 CACNL1A CACNL1A | 37 |
Migraine | Familial hemiplegic migraine 1 Familial hemiplegic migraine 2 | Ca Na | CACNL1A SCN1A | 34 |
Epilepsy | Benign neonatal familial convulsions Generalized epilepsy with febrile convulsions plus | K Na | KCNQ2, KCNQ3 SCN1B | 31 |
Periodic paralysis | Hyperkalemic periodic paralysis Hypokalemic periodic paralysis | Na Ca | SCN4A CACNL1A3 | 56 |
Myotonia | Myotonia congenita Paramyotonia congenita | Cl Na | CLCN1 SCN4A | 56 |
Deafness | Jervell and Lange-Nielsen syndrome (deafness, prolonged QT interval, and arrhythmia) | K | KCNQ1, KCNE1 | 29 |
Autosomal dominant progressive deafness | K | KCNQ4 | ||
Autoimmune | ||||
Paraneoplastic | Limbic encephalitis Acquired neuromyotonia Cerebellar ataxia Lambert-Eaton syndrome | Kv1 Kv1 Ca (P/Q type) Ca (P/Q type) | — — — — | 50 |
Whereas the specific clinical manifestations of channelopathies are quite variable, one common feature is that manifestations tend to be intermittent or paroxysmal, such as occurs in epilepsy, migraine, ataxia, myotonia, or periodic paralysis. Exceptions are clinically progressive channel disorders such as autosomal dominant hearing impairment. The genetic channelopathies identified to date are all uncommon disorders caused by obvious mutations in channel genes. As the full repertoire of human ion channels and related proteins is identified, it is likely that additional channelopathies will be discovered. In addition to rare disorders that result from obvious mutations, it is also likely that less penetrant allelic variations in channel genes or in their pattern of expression might underlie susceptibility to some apparently sporadic forms of epilepsy, migraine, or other disorders. For example, mutations in the potassium channel gene Kir2.6 have been found in many individuals with thyrotoxic hypokalemic periodic paralysis, a disorder similar to hypokalemic periodic paralysis but precipitated by stress from thyrotoxicosis or carbohydrate loading.
Synaptic neurotransmission is the predominant means by which neurons communicate with each other. Classic neurotransmitters are synthesized in the presynaptic region of the nerve terminal; stored in vesicles; and released into the synaptic cleft, where they bind to receptors on the postsynaptic cell. Secreted neurotransmitters are eliminated by reuptake into the presynaptic neuron (or glia), by diffusion away from the synaptic cleft, and/or by specific inactivation. In addition to the classic neurotransmitters, many neuropeptides have been identified as definite or probable neurotransmitters; these include substance P, neurotensin, enkephalins, β-endorphin, histamine, vasoactive intestinal polypeptide, cholecystokinin, neuropeptide Y, and somatostatin. Peptide neurotransmitters are synthesized in the cell body rather than the nerve terminal and may colocalize with classic neurotransmitters in single neurons. A number of neuropeptides are important in pain modulation including substance P and calcitonin gene-related peptide (CGRP), which causes migraine-like headaches in patients. As a consequence, CGRP receptor antagonists have been developed and shown to be effective in treating migraine headaches. Nitric oxide and carbon monoxide are gases that appear also to function as neurotransmitters, in part by signaling in a retrograde fashion from the postsynaptic to the presynaptic cell.
Neurotransmitters modulate the function of postsynaptic cells by binding to specific neurotransmitter receptors, of which there are two major types. Ionotropic receptors are direct ion channels that open after engagement by the neurotransmitter. Metabotropic receptors interact with G proteins, stimulating production of second messengers and activating protein kinases, which modulate a variety of cellular events. Ionotropic receptors are multiple subunit structures, whereas metabotropic receptors are composed of single subunits only. One important difference between ionotropic and metabotropic receptors is that the kinetics of ionotropic receptor effects are fast (generally <1 ms) because neurotransmitter binding directly alters the electrical properties of the postsynaptic cell, whereas metabotropic receptors function over longer time periods. These different properties contribute to the potential for selective and finely modulated signaling by neurotransmitters.
Neurotransmitter systems are perturbed in a large number of clinical disorders, several of which are highlighted in Table 30-2. One example is the involvement of dopaminergic neurons originating in the substantia nigra of the midbrain and projecting to the striatum (nigrostriatal pathway) in Parkinson’s disease and in heroin addicts after the ingestion of the toxin MPTP (1-methyl-4-phenyl-1,2,5,6-tetrahydropyridine).
NEUROTRANSMITTER | ANATOMY | CLINICAL ASPECTS |
---|---|---|
Acetylcholine (ACh) | Motor neurons in spinal cord → neuromuscular junction | Acetylcholinesterases (nerve gases) Myasthenia gravis (antibodies to ACh receptor) Congenital myasthenic syndromes (mutations in ACh receptor subunits) Lambert-Eaton syndrome (antibodies to Ca channels impair ACh release) Botulism (toxin disrupts ACh release by exocytosis) |
Basal forebrain → widespread cortex | Alzheimer disease (selective cell death) Autosomal dominant frontal lobe epilepsy (mutations in CNS ACh receptor) | |
Interneurons in striatum | Parkinson’s disease (tremor) | |
Autonomic nervous system (preganglionic and postganglionic parasympathetic; preganglionic sympathetic) | ||
Dopamine | Substantia nigra → striatum (nigrostriatal pathway) Substantia nigra → limbic system and widespread cortex Arcuate nucleus of hypothalamus → anterior pituitary (via portal veins) | Parkinson’s disease (selective cell death) MPTP parkinsonism (toxin transported into neurons) Addiction, behavioral disorders Inhibits prolactin secretion |
Norepinephrine (NE) | Locus coeruleus (pons) → limbic system, hypothalamus, cortex Medulla → locus coeruleus, spinal cord Postganglionic neurons of sympathetic nervous system | Mood disorders (MAOA inhibitors and tricyclics increase NE and improve depression) Anxiety Orthostatic tachycardia syndrome (mutations in NE transporter) |
Serotonin | Pontine raphe nuclei → widespread projections Medulla/pons → dorsal horn of spinal cord | Mood disorders (SSRIs improve depression) Migraine pain pathway Pain pathway |
γ-Aminobutyric acid (GABA) | Major inhibitory neurotransmitter in brain; widespread cortical interneurons and long projection pathways | Stiff person syndrome (antibodies to glutamic acid decarboxylase, the biosynthetic enzyme for GABA) Epilepsy (gabapentin and valproic acid increase GABA) |
Glycine | Major inhibitory neurotransmitter in spinal cord | Spasticity Hyperekplexia (myoclonic startle syndrome) due to mutations in glycine receptor |
Glutamate | Major excitatory neurotransmitter; located throughout CNS, including cortical pyramidal cells | Seizures due to ingestion of domoic acid (a glutamate analogue) Rasmussen’s encephalitis (antibody against glutamate receptor 3) Excitotoxic cell death |
A second important dopaminergic system arising in the midbrain is the mediocorticolimbic pathway, which is implicated in the pathogenesis of addictive behaviors including drug reward. Its key components include the midbrain ventral tegmental area (VTA), median forebrain bundle, and nucleus accumbens (see Fig. 60-2). The cholinergic pathway originating in the nucleus basalis of Meynert plays a role in memory function in Alzheimer’s disease.
Addictive drugs share the property of increasing dopamine release in the nucleus accumbens (Chap. 60). Amphetamine increases intracellular release of dopamine from vesicles and reverses transport of dopamine through the dopamine transporters. Patients prone to addiction show increased activation of the nucleus accumbens following administration of amphetamine. Cocaine binds to dopamine transporters and inhibits dopamine reuptake. Ethanol inhibits inhibitory neurons in the VTA, leading to increased dopamine release in the nucleus accumbens. Opioids also disinhibit these dopaminergic neurons by binding to μ receptors expressed by γ-aminobutyric acid (GABA)–containing interneurons in the VTA. Nicotine increases dopamine release by activating nicotinic acetylcholine receptors on cell bodies and nerve terminals of dopaminergic VTA neurons. Tetrahydrocannabinol, the active ingredient of cannabis, also increases dopamine levels in the nucleus accumbens. Blockade of dopamine in the nucleus accumbens can terminate the rewarding effects of addictive drugs.
Not all cell-to-cell communication in the nervous system occurs via neurotransmission. Gap junctions provide for direct neuron-neuron electrical conduction and also create openings for the diffusion of ions and metabolites between cells. In addition to neurons, gap junctions are also widespread in glia, creating a syncytium that protects neurons by removing glutamate and potassium from the extracellular environment. Gap junctions consist of membrane-spanning proteins, termed connexins, that pair across adjacent cells. Mechanisms that involve gap junctions have been related to a variety of neurologic disorders. Mutations in connexin 32, a gap junction protein expressed by Schwann cells, are responsible for the X-linked form of CMT disease (Chap. 53). Mutations in either of two gap junction proteins expressed in the inner ear—connexin 26 and connexin 31—result in autosomal dominant progressive hearing loss (Chap. 29). Glial calcium waves mediated through gap junctions also appear to explain the phenomenon of spreading depression associated with migraine auras and the march of epileptic discharges. Spreading depression is a neural response that follows a variety of different stimuli and is characterized by a circumferentially expanding negative potential that propagates at a characteristic speed of 20 m/s and is associated with an increase in extracellular potassium.
The fundamental issue of how memory, learning, and thinking are encoded in the nervous system is likely to be clarified by identifying the signaling pathways involved in neuronal differentiation, axon guidance, and synapse formation, and by understanding how these pathways are modulated by experience. Many families of transcription factors, each comprising multiple individual components, are expressed in the nervous system. Elucidation of these signaling pathways has already begun to provide insights into the cause of a variety of neurologic disorders, including inherited disorders of cognition such as X-linked mental retardation. This problem affects ~1 in 500 males, and linkage studies in different families suggest that as many as 60 different X-chromosome-encoded genes may be responsible. The formation of RNA-DNA duplexes that block transcription has also been observed with the CGG repeat expansions that occur in fragile X gene-associated mental retardation. Rett’s syndrome, a common cause of (dominant) X-linked progressive mental retardation in females, is due to a mutation in a gene (MECP2) encoding a DNA-binding protein involved in transcriptional repression. Because the X chromosome comprises only ~3% of germline DNA, then by extrapolation, the number of genes that potentially contribute to clinical disorders affecting intelligence in humans must be potentially very large. As discussed below, there is increasing evidence that abnormal gene transcription may play a role in neurodegenerative diseases, such as Huntington’s disease, in which proteins with polyglutamine expansions bind to and sequester transcription factors. A critical transcription factor for neuronal survival is CREB (cyclic adenosine monophosphate responsive element-binding) protein, which also plays an important role in memory in the hippocampus. The regulatory gene repressor element 1-silencing transcription factor (REST) coordinates the expression of neuroprotective stress genes during normal aging. It turns off genes involved in cell death and pathology and boosts protective factors. High levels of REST are associated with normal cognition even in the presence of both amyloid plaques and neurofibrillary tangles. Although REST increases with normal aging, it fails to increase in the nucleus in patients with Alzheimer’s disease and is found clumped with amyloid in autophagosomes.
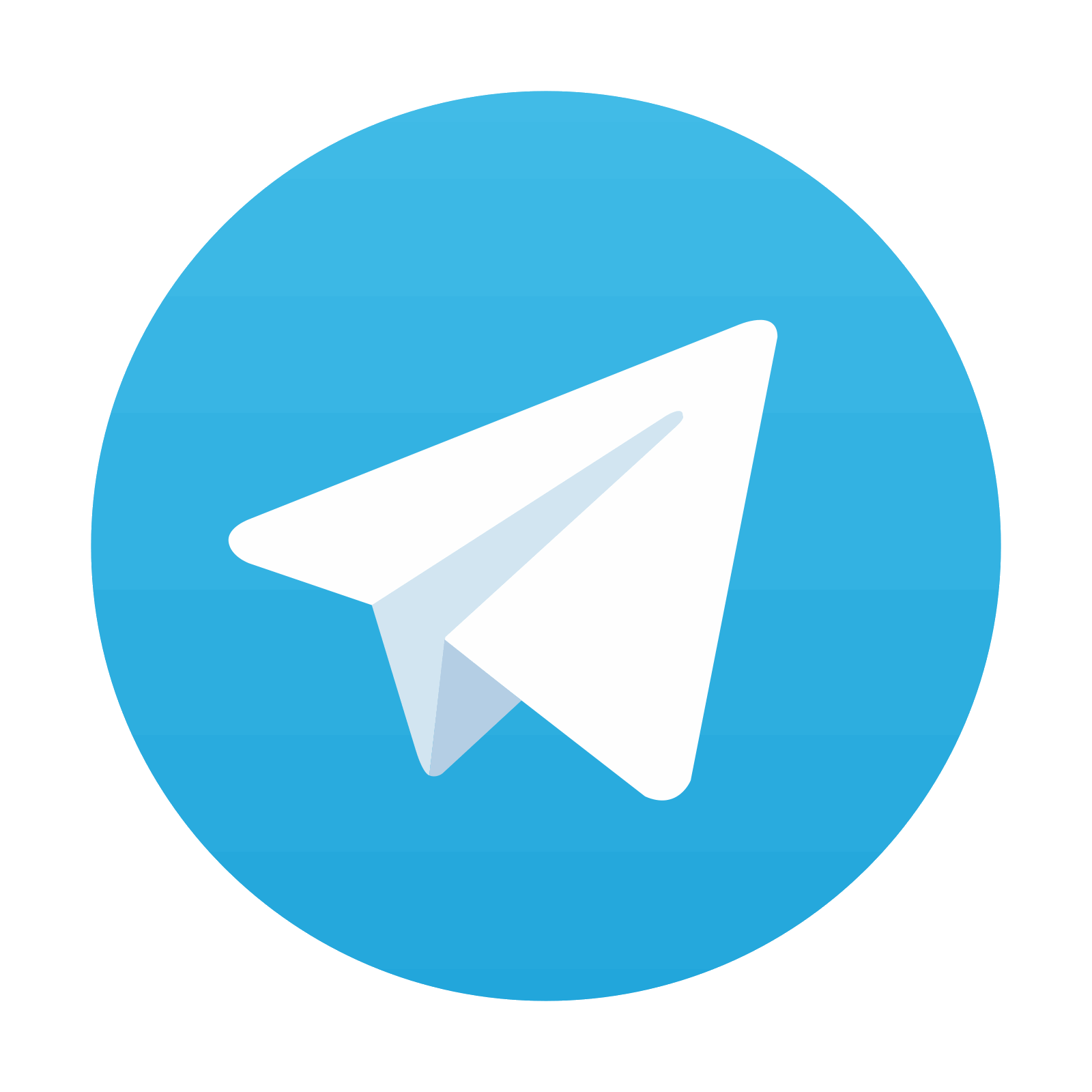
Stay updated, free articles. Join our Telegram channel
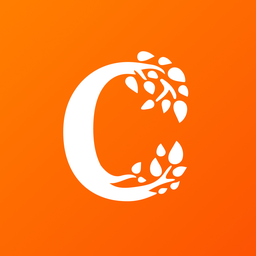
Full access? Get Clinical Tree
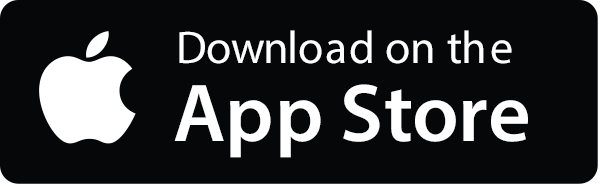
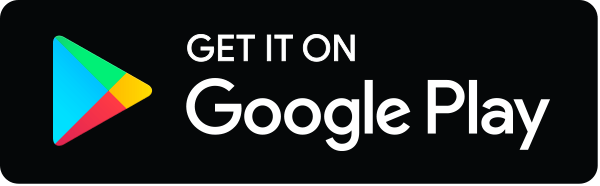