Abbreviations
APRIL
a proliferation-inducing ligand
ASIA
American Spine Injury Association
AZGP
zinc alpha 2 glycoprotein
B2M
β2 microglobulin
BAFF
B-cell activating factor
BCMA
B-cell maturation antigen
BSCB
blood spinal cord barrier
CathD
cathepsin D
CCL2
chemokine ligand 2
CD95L
CD95 (Fas/APO-1)-ligand
CNS
central nervous system
CSF
cerebral spinal fluid
DEmiRNAs
differently expressed miRNAs
EAE
experimental autoimmune encephalomyelitis
ENO2
enolase 2
EV
extracellular vesicle
FGF
fibroblast growth factor
GBM
glioblastoma
GDNF
glial cell-derived neurotrophic factor
GFAP
glial fibrillary-acidic protein
HMGB1
high mobility group 1 box
HP
haptoglobin
IGF
insulin-like growth factor
IL
interleukin
INF
interferon
IP
C-X-C motif chemokine ligand 10
MBP
myelin basic protein
MCP
monocyte chemotactic protein
MIF
macrophage migration inhibitor
miRNAs
microRNAs
MMPs
metalloproteinases
MS
multiple sclerosis
NFL
neurofilaments
NSE
neuron-specific enolase
PEA15
phosphoprotein enriched in astrocytes 15
pNF-H
phosphorylated form of neurofilament heavy chain
S100β
glial-specific calcium-binding β protein
SBDP
spectrin breakdown products
SCI
spinal cord injury
TF
transferrin
TGF
growth factor transformer
TPI
triosephosphate isomerase
UCH-L1
ubiquitin carboxy-terminal hydrolase L1
VEGF
vascular endothelial growth factor
Acknowledgments
This work was supported by the National Institute for Translational Neuroscience (INNT) of the Ministry of Science and Technology ; the Brazilian Federal Agency for the Support and Evaluation of Graduate Education (CAPES) of the Ministry of Education ; the National Council for Scientific and Technological Development (CNPq); the Carlos Chagas Filho Research Support Foundation (FAPERJ); and the Ary Frauzino Foundation for Cancer Research.
Introduction
Spinal cord injury (SCI) is a broad term that includes changeable grades of neurological deficits and is still considered an incurable condition. In spite of substantial advances in surgical and medical treatment, just around 1% of SCI patients experience complete healing. Unfortunately, almost 45% of the them suffer from serious neurological loss, which includes incomplete or complete tetraplegia, with or without respiratory compromise ( ). Therefore, SCI usually has devastating vocational, social, and physical implications for patients and caregivers ( ).
The patient’s outcome after initial SCI is still very difficult to predict, because the tools we have today for both evaluating the gravity of spinal cord tissue destruction and better assessing the recovery of SCI patients are still restricted ( ). Imaging examinations of the spinal cord cannot accurately predict the prognosis of the disease. Computed tomography shows only fractures or dislocations, while magnetic resonance imaging is not highly sensitive to edema of the spinal cord, be it related or not to SCI. Furthermore, laboratory tests of blood biochemistry such as lactate dehydrogenase and protein kinase C are not specific for SCI, as other non-neurological damage can also cause changes in blood parameters, having, therefore, no clinical application ( ). There’s no doubt that the sooner we evaluate the extent of the lesion and start treating the patient, the better the outcome is. Nowadays, the best predictive value of neurological assessment of the SCI patient is defined by typical clinical neurological examinations during the patient’s first evaluation ( ). So the identification of biomarkers that can predict lesions is crucial to helping assess damage severity, prognosis, and therapeutic outcomes ( ).
In this chapter, we will highlight the most recent research on the expression of biomarkers in the pathophysiology of the SCI and try to correlate to diagnosis and prognosis.
Pathophysiology of SCI
It is well known that SCI occurs in two mechanisms that progress through three stages. Both mechanisms that lead to acute SCI are now very well characterized and recognized as primary (mechanical) and secondary injuries. The primary injury is caused by the initial mechanical trauma of surrounding tissues, followed by contusion and compression of the spinal cord, which induces damage to nerve cells, blood vessels, and myelin ( ). The secondary lesion is triggered by the primary lesion and is classified in three phases, namely the acute phase, the sub-acute (or intermediate) phase, and the chronic phase, according to the pathomechanism and post-injury time ( ; ).
The acute stage begins with the mechanical injury and extends to the first 48 h after the initial traumatic injury. In this stage, the patient develops some neurological deficits directly associated with the mechanical injury. This is when innumerable pathophysiological processes begin, leading to the initial inflammatory response to the injury and causing patients to develop “spinal shock” ( ; ). It is well established that, at this stage, crucial events are induced by vascular rupture, ischemia, and hemorrhage by the action of inflammatory growth factors which affects neurons and glial cells, including excessive production of free radicals, ionic dysregulation, inflammatory response, and excitotoxicity, inducing pathological changes ( ; ). At this stage, primary care should be provided for the patients, including protection of the airways, maintenance of the respiratory function, and use of hemodynamic support ( ).
It is, however, often difficult to define the baseline severity of the injury, as the patient is often in an unstable clinical condition due to spinal shock ( ). In order to reduce inflammation and neurological deficits, some centers use high-dose corticosteroids in the first 8 h after the injury, following the NASCIS II protocol ( ). Nevertheless, damage to glial cells, neurons and oligodendrocytes caused by the presence of excessive amounts of excitatory neurotransmitters (glutamate, aspartate) cannot be prevented. Furthermore, neurons die during all phases of the injury due to necrosis and astrocytes. Neurons and oligodendrocytes also die due to apoptotic mechanisms ( ).
The secondary or sub-acute stage lasts up to 2 weeks after the damage. At this stage, the patient usually recovers from the initial spine shock. However, neurological loss as well as other potential complications associated with the original trauma continue, as nerve cell dysfunction and blood supply problems derived from the acute stage can get even worse ( ). In this phase, reactive gliosis increases both the content of glial fibrillary-acidic protein (GFAP) and the size of astrocytes to form the glial scar, In this phase, an intense inflammatory response begins, whereby phagocytic cells are recruited ( ; ). The glial scar, with its firmly fused processes, forms an inhibitory agglomerate in the form of a mesh that plays a fundamental role in interrupting axonal regeneration ( ). However, despite limiting regeneration after an injury to the central nervous system (CNS), the uncontrolled proliferation of reactive astrocytes suppresses the formation of aberrant synapses at the injury site and also contributes to both the restoration of the integrity of the blood spinal cord barrier (BSCB) and the reconstruction of microenvironment homeostasis ( ). This mechanism is important for the removal of edemas, in addition to reducing the infiltration of cells of the immune system, helping, thus to limit the spread of the lesion ( ).
The chronic SCI phase, whose characterization requires further investigation, follows the sub-acute SCI phase. At this stage, patients may experience partial neurological recovery, which will depend on the extent of the lesion and the general condition of each patient. In addition, patients may also have maturation of adaptive mechanisms or the onset of more delayed neurological symptoms such as urinary bladder dysfunction, neuropathic pain, lipodystrophy, musculoskeletal atrophy, dysautonomia, and abnormal skeletal postures ( ). This phase may prolong from days to years after the shock, and is characterized by Wallerian degeneration, apoptosis and scarring that cause functional impairment ( ; ). At this stage, it is also normal for patients to develop syringomyelia * due to the formation of the glial scar. Therapeutic strategies generally focus on increasing the regeneration of damaged axons and remyelination through different mechanisms, whether through pharmacological methods or cell therapy ( ).
Biomarkers of SCI
First, it is important to define the word biomarker *. Biomarkers, also known as “biological markers,” are related to a wide sub-category of medical signs which can be measured with precision and reproducibility. Thus, biomarkers are, for purposes of interpretation, quantifiable results of biological mechanisms ( ). A biomarker of tissue injury should ideally be abundant, preferably (or exclusively) produced in the tissue of interest, and to be naturally present in small concentrations in blood and other body fluids ( ; ). It is also important to have good biomarkers that can contribute as a measure of valuable therapeutic responses. They are highly valuable in the decision-making process of seeking additional clinical evaluation of a drug, and in helping to define critical parameters such as the intervention time window, drug dose and monitoring schedule for a clinical trial. It is also important to have good biomarkers that are acutely related to biochemical changes made or sustained during all phases of SCI. It is finally very important to validate all the potential biomarkers candidates.
Currently, there are neither drugs for treatment nor specific laboratory tests for the diagnosis of SCI. Therefore, in order to determine the extent of SCI as soon as possible, it is urgent to further the discovery and use of specific biomarkers for SCI. This could help guide doctors and researchers toward the discovery of a new object of intervention that can help prevent and decrease disability resulting from SCI.
SCI in the acute stage
In the acute stage, there is a change in the permeability of the BSCB, due to the formation of glial scarring and the disturbance of endothelial cells. These cells secrete various inflammatory cytokines and some are currently being investigated as potential SCI biomarkers. High concentrations of interleukin-6 (IL-6), interleukin-8 (IL-8), and monocyte chemotactic protein (MCP)-1 (also known as chemokine ligand 2 (CC motif) (CCL2)) have been expressed in CSF. They have been shown to be dependent on the severity of the injury, when patients presented with American Spinal Injury Association grade A (ASIA A) injury had higher levels of these cytokines compared to ASIA B and C patients ( ).
Recently, a study with 15 ASIA A and B patients demonstrated that IL-10, IL-1rα, IL-9, IL-16, and IL-18 were positively regulated 24 h after SCI, and that interferon-gamma (INF-γ) and IL-13 have been downregulated ( ). Upregulation of the high mobility group 1 box (HMGB1) and the inflammatory cytokine macrophage migration inhibitor (MIF) factor were also identified, both occurring regardless of the degree or severity of injury ( ). Several studies also analyzed the transient expression of some proteins during the acute phase. Some structural proteins in glial cells and neurons are usually modulated in the CSF and in the serum of patients with acute SCI. Proteins such as GFAP, Phosphorylated Neurofilament Heavy (pNF-H), light chains of neurofilaments (NFL, 68 kDa), neuron-specific enolase (NSE), glial-specific calcium-binding β protein (S100β), tau, ubiquitin carboxy-terminal hydrolase L1 (UCH-L1), αII spectrin breakdown products (SBDP), myelin basic protein (MBP), transferrin, cathepsin D, triosephosphate isomerase-1, and astrocytic phosphoprotein PEA-15 were analyzed in patients with acute SCI ( ; ; ; ).
GFAP is an astrocyte-specific intermediate filament protein associated with glial scar formation, and astrocyte protein S100β in adults is generally elevated due to damage to the nervous system. It has been shown that both GFAP and S100β are present at higher levels in CSF and serum 24 h after injury, depending on severity, and that both can serve as a prognostic biomarker ( ; ). Inverse results were observed with the tau protein, which is an intracellular neuron protein involved in the stabilization of microtubules. A negative correlation was observed between CSF and serum tau concentrations and the severity of spinal cord injury 24 h after the injury, suggesting that tau may score as a prognostic biomarker for neurological improvement after acute spinal cord injury ( ; ).
NSE, p-NF-H, NFL have been found to be elevated in the serum and CSF of patients with acute SCI. However, when p-NF-H decreases to normal values, it allows the prediction of a favorable outcome ( ). UCH-L1, SBDP, MBP have also been found to be transiently elevated in the CSF of patients with SCI and show a correlation between severity and recovery ( ) (see Table 1 ).
Expression in CSF | Cytokines | Proteins |
---|---|---|
Upregulated | IL-6, IL-8, IL-10, IL-1rα, IL-9, IL-16, IL-18MCP-1 (CCL2), HMGB1, MIF | GFAP, S100β, NSE, p-NF-H, NFL, UCH-L1, SBDP, MBP |
Downregulated | INF-γ, IL-13 |
SCI in the sub-acute stage
In the sub-acute stage, during the formation of the glial scar *, astrocytes increase the expression of not only GFAP, but also of nestin and vimentin. It has also been reported that, at this stage, astrocytes also secrete various cytokines* and growth factors such as vascular endothelial growth factor (VEGF), fibroblast growth factor (FGF)-β, glial cell-derived neurotrophic factor (GDNF), and growth factor transformer (TGF)-β, which are speculated to promote in later stages the differentiation, proliferation and migration of oligodendrocyte precursor cells. TGF-β1 is suggested to be implicated in scar formation after spinal cord injury ( ; ).
Recently, a study showed that patients with SCI had a decrease in both serum TGF-β1, an insulin-like growth factor 1 (IGF-1), and in sCD95L, but there was an upregulation 12 weeks after the injury correlated with the absence of neurological recovery ( ).
CD95(Fas/APO-1)-ligand (CD95L) protein during the sub-acute phase is cleaved and a portion of it is released into the peripheral blood. This protein induces apoptosis in CD95-sensitive cells, especially at the sub-acute stages when apoptotic activity increases. This protein has the potential of acting as a biomarker at the sub-acute stage, and mainly of indicating the apoptotic effect destructive to the spinal cord tissue and the consequent neurological loss ( ). At this stage, the phagocytic inflammatory cells normally release cytokines that could be used as biomarkers. One of those cytokines is IL-1β, which has shown a significant fall at 1 and 4 weeks post injury in patients who experienced less progress ( ). Several metalloproteinases (MMPs) are upregulated during the sub-acute phase, and specially MMP-8 and -9, which have been pointed as a useful indicator for recovery potential ( ; ). The C-X-C motif chemokine ligand 10 (IP-10) was found increased in serum at 3–6 h after SCI. However, there was a peak after 7 days of trauma at the sub-acute phase ( ). Other proteins were pointed as potential biomarkers in the sub-acute phase, among them transferrin (TF), cathepsin D (CathD), triosephosphate isomerase-1 (TPI-1), phosphoprotein enriched in astrocytes 15 (PEA15), zinc alpha 2 glycoprotein (AZGP) and Haptoglobin (HP). They were found in human CSF from SCI patients, whose AZGP and HP protein levels decline in the CSF as the secondary injury process progresses ( ; ) (see Table 2 ).
Expression in CSF | Metalloproteinase | Proteins | Growth factors | Cytokines |
---|---|---|---|---|
Upregulated | MMP-8 and 9 | Nestin, Vimentin, TF, CathD, TPI-1, PEA15 | GFAP, VEGF, FGF-β, GDNF, TGF-β1 a , IGF-1 a , CD95L a | IP-10 |
Downregulated | AZGP, HP |
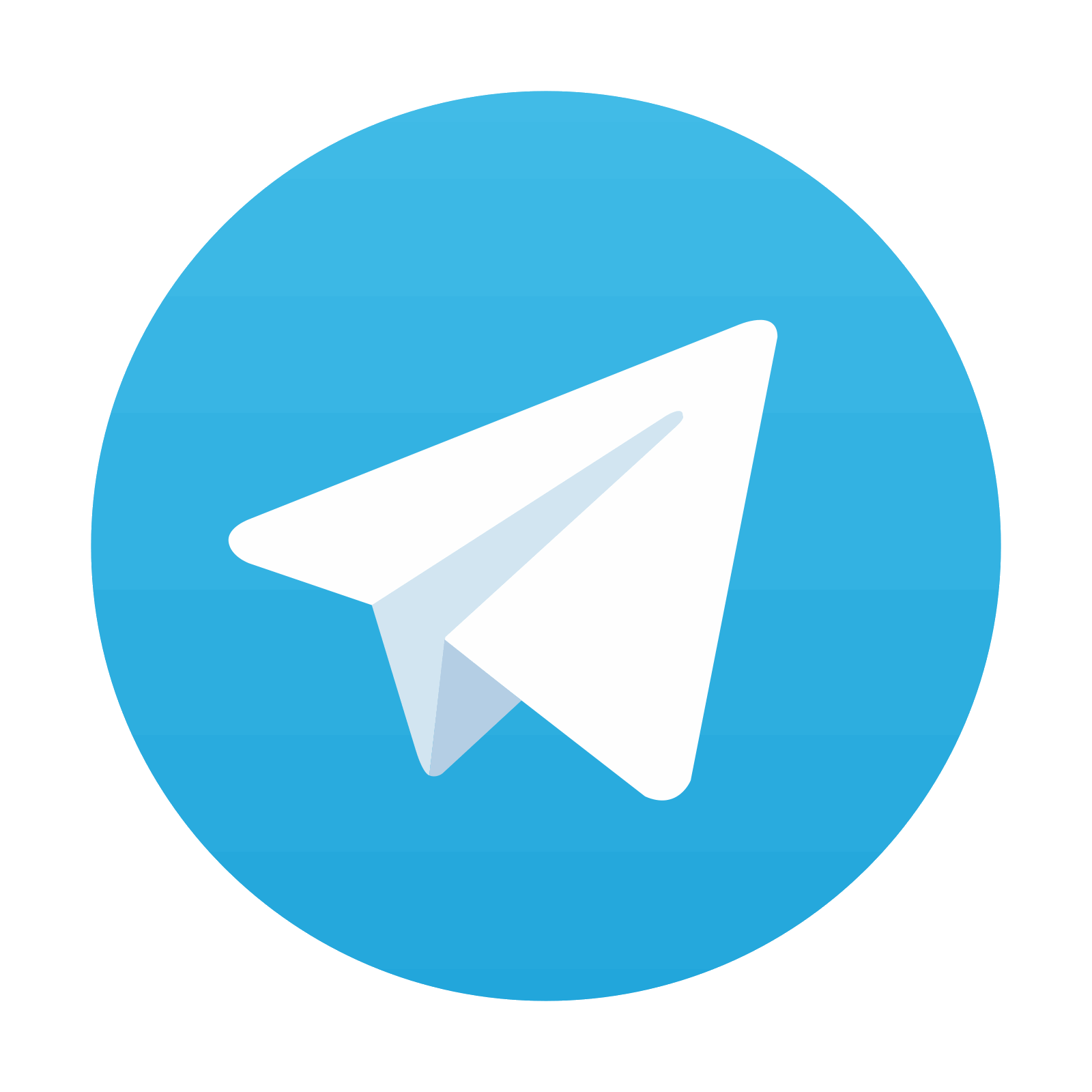
Stay updated, free articles. Join our Telegram channel
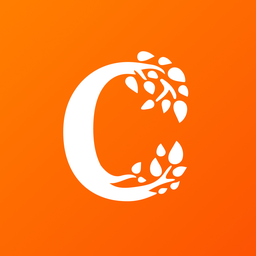
Full access? Get Clinical Tree
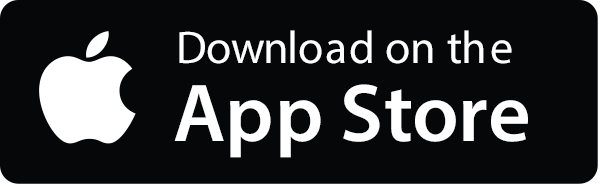
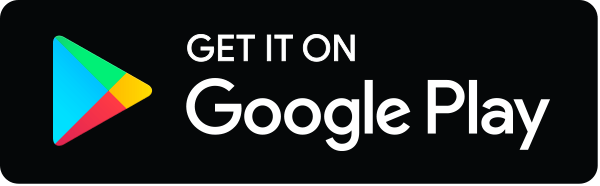
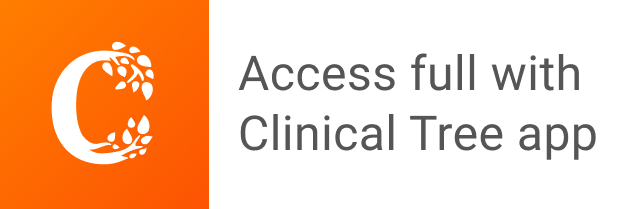