Fig. 7.1
(a) A simplified diagram of the tight blood–brain barrier (BBB) which separates blood from the extracellular fluid (ECF) of brain parenchyma. Very tight cell membranes prevent proteins from the cytosol diffusing into the ECF. The less tight blood–CSF barrier (BCB) allows substances to diffuse from the blood into the CSF. (b) Breakdown of the blood–CSF barrier results in leakage of albumin from blood into CSF. (c) Cellular death following brain damage leads to disintegration of the cellular membrane. Biomarkers leak from the cytoplasm into the adjacent ECF (From ECF these biomarkers then equilibrate with the CSF. Figure reproduced with permission from Maney Publishing, reference [22] www.maney.co.uk/journals/ner and www.ingentaconnect.com/content/maney/nres)
The blood–brain barrier (BBB) acts as a filter preventing the unselective diffusion of compounds into the human brain (Fig. 7.1). To be precise, the anatomically defined BBB needs to be distinguished from the functionally defined blood–CSF barrier (BCB). The BCB is a sieve permitting small substances to diffuse from the blood into the CSF [21]. At time of writing, the gold standard for assessment of the BBB/BCB function is still based on the measurement of albumin in the CSF and serum [23]. Albumin is not produced in the brain but by the liver. Therefore, all albumin measured in the CSF must originate from the blood [24]. If the BBB/BCB is intact, only a small amount of albumin can diffuse into the CSF (normal range ≈144–336 mg/L). In normal conditions, the CSF to serum albumin quotient is smaller than 0.0074 [23]. With breakdown of the BCB, serum albumin leaks into the CSF (Fig. 7.1b), the CSF albumin rises, and the CSF to serum albumin quotient increases.
7.2.4 Cell-Type-Specific Biomarkers
In MS, disintegration of the axonal membrane causes release of cytoplasmic contents from injured axons into surrounding extracellular fluid (ECF) of the human CNS [25]. These substances diffuse from the brain ECF into the CSF and blood from where they can be sampled and quantified (Fig. 7.2). Of note, some biomarkers are only expressed by certain cell types. They are called cell-type-specific biomarkers. The measurement of cell-type-specific biomarkers may indirectly permit an estimation of the degree of specific cellular injury occurring, e.g., axonal loss, and may thus be useful in prognostication concerning disease course.


Fig. 7.2
The evolution of oligoclonal band diagnostics in MS
Table 7.1 summarizes cell-type-specific and other biomarkers of relevance to progressive MS.
Table 7.1
Potential and established CSF biomarkers and their cellular sources. CSF biomarkers for the neuron and axon are of particular interest for disease progression in MS
CSF biomarker | Neuron/axon | Astrocyte | Microglia | Oligodendrocyte | Choroid plexus |
---|---|---|---|---|---|
14-3-3γ | ++ | + | + | + | |
ABP | + | ||||
AQP1 and AQP4 | + | + | |||
AD7c-NTP | + | ||||
Albumin | |||||
α-spectrin | + | + | + | + | + |
α-(1)BG | + | ||||
α- internexin | + | ||||
ApoE | + | ||||
β-tubulin | + | ||||
β-2-microglobulin | + | ||||
β-trace | + | (+) | |||
Bri2-23 | + | ||||
Chitinase 3-like 1 | ? | ||||
Chromagrannins A & B | + | ||||
Clusterin | + | + | + | + | |
Cystatin C | + | + | |||
EDG-8 | + | ||||
FABPs | + | + | + | + | |
FFA | + | + | + | + | |
Ferritin | + | ||||
Fetuin-A | + | ||||
GFAP | + | ||||
Glucose | + | + | + | + | |
Glutamate | + | + | + | + | |
HK6 | + | ||||
HNE | + | + | + | + | |
Hypocretin-1 | + | ||||
Isoprostanes | + | + | + | + | |
Lactate | + | + | + | + | |
MAG | + | ||||
MBP | + | ||||
MDA | + | + | + | + | |
MOBP | + | ||||
MOG | + | ||||
NAA | ++ | + | + | + | |
NCAM | + | + | |||
NOx | + | + | + | ||
NSE | + | ||||
Neurotrophins | + | + | + | + | |
Nf | ++ | ||||
OMgp | + | ||||
Osteopontin | + | + | + | + | |
PLP | + | ||||
PrPc | + | ||||
Pyruvate | + | + | + | + | |
S100B | ++ | + | |||
Secretogranins | + | + | + | ||
SFas (sCD95) | + | ||||
Tau | + | + | + | + | |
Transferrin | + | + | |||
Transthyretin | + | ||||
Ubiquitin | + | + | + | + | |
UCHL-1 | ++ | ||||
Vimentin | + | + | + |
7.2.5 Challenges for Developing Biomarkers in MS
Pathological disease heterogeneity, highly variable clinical courses, subclinical disease activity, and the fluctuating clinical course of acute relapses on top of preexisting damage are all factors which increase the challenge of developing biomarkers for MS. Certain cell-type-specific biomarkers may not be useful at the delineation of specific pathological processes. For example, the glial biomarkers S100B or glial fibrillary acidic protein (GFAP) will be increased following acute relapse because of astrocyte activation as well as after formation of a glial scar later in the disease course. Even more difficult to interpret may be biomarkers indicating systemic inflammation independent of whether or not there are acute MS lesions forming in the brain.
In contrast, biomarkers specific for the axonal compartment are likely to indicate acute damage to the structure pathologically linked to disability progression [4, 5]. To add value, a biomarker should improve on the clinical prognostic accuracy. At disease onset, either the optic nerve, spinal cord, or brainstem are affected in 85% of cases [26, 27]. The risk for developing MS is higher with spinal cord involvement compared to isolated optic nerve pathology, yet not all patients presenting with optic neuritis (ON) or transverse myelitis (TM) develop MS [1, 28]. This implies that a biomarker result cannot be interpreted in isolation, but needs to be interpreted in the context of the overall clinical assessment.
7.3 CSF Biomarkers in MS
7.3.1 Oligoclonal Bands
There is no diagnostic biomarker for MS. A reasonably sensitive biomarker is the demonstration of intrathecal IgG synthesis. Elvin Kabat, a neurologist at the Neurological Institute of the College of Physicians and Surgeons at Columbia University in New York, was probably the first to make use of Arne Tiselius’ electrophoresis to investigate human CSF. In 1942, he required about 70 mL CSF for one analysis (about half of total CSF volume!; for comparison, today we only require about 2–4 μL). Kabat’s key observation was an increase of globulin in the CSF, not seen in the serum. The earliest example of CSF protein electrophoresis showing a visible increase of CSF globulin in patients with MS is from H. Bauer in 1953 (Fig. 7.2).
Since then, methodological refinements, in particular the development of isoelectric focusing (IEF) on agarose gels followed by immunoblotting, have improved the resolution [29]. We now know that the increase of globulin observed by Kabat in the CSF of patients with MS is due to intrathecally produced oligoclonal IgG (Fig. 7.2). Standardized basic CSF analysis is required in order to maximize the diagnostic specificity for OCBs in the differential diagnosis of MS.
There are six core points to be considered when analyzing CSF as part of the diagnostic work-up for MS:
1.
OCBs are regarded as an extension of the clinical assessment
2.
CSF cytology:
A high red blood cell count (5 × 109/L to 7 × 109/L) in the absence of bilirubin (assessed by spectrophotometry) suggests a traumatic tap. This may render other quantitative tests uninterpretable.
A mildly raised white cell count (>5 × 106/L) may be found in up to 34% of patients with MS [30].
A high white cell count (>50 × 106/L) is unusual in MS.
3.
CSF glucose: the CSF/serum ratio should be >0.4; low CSF glucose levels suggest an infectious process [31].
4.
CSF total protein: a very high CSF total protein content (>1 g/L) suggests an infectious or neoplastic process. High CSF total protein is occasionally seen in patients with CIDP who also have central demyelination.
5.
CSF/serum albumin quotient: allows assessment of the integrity of the blood–CSF barrier and is the basis for quantitative models of intrathecal immunoglobulins.
6.
CSF lactate: an increase in CSF lactate (>2.4 nmol/L) is unusual in MS and may suggest mitochondrial or infectious pathology.
Good clinical selection and a basic CSF program help to minimize pre-analytical pitfalls leading to a false-positive or false-negative CSF OCB results. Five fundamental keys to understanding OCBs are: in normal CSF, all IgG comes from the blood by passive diffusion; in normal CSF and serum, IgG is polyclonal; oligoclonal bands in blood give a mirror pattern in CSF; local synthesis is present when there are bands in the CSF that are absent from the serum; oligoclonal bands are (generally) a sign of pathology.
In his 2003 review, Keren showed that loss of analytical quality led to reported frequencies of CSF OCBs in MS in the range of 45–77% [32]. A sensitivity of 45% is clearly not acceptable and contrasts with sensitivity levels found in large studies using isoelectric focusing (Table 7.2). Therefore, recommendations for CSF analysis have been developed [31].
The enormous diversity of IgG antibodies is achieved by IgG gene rearrangement (somatic hypermutation) during B-cell development (clonal expansion) [36]. Antibody diversity is needed because of the wide-ranging and changing pathogenic threat. For this reason, any systemic infection triggers an oligoclonal response. Consequently, a large number of B-cells are recruited and stimulated by cytokines. Polyclonal IgG responses in the serum are probably the result of this massive cytokine stimulation, resulting in activity of B-cells. Each of these B-cell clones produces a slightly differently composed IgG molecule targeted at the potential antigen threat. Over time, particularly effective clones may be selected. This process is not fully understood and modification of B-cells in the germinal centers plays a role alongside somatic mutations and affinity maturation.
In the CNS, only a small number of B-cell clones are present and hence the intrathecal B-cell immune response can only ever be oligoclonal. Further development of analytical techniques may reveal more about the different clones and their immunoglobulins.
From a biological point of view, there appears to be a continuum from the systemic polyclonal immune response to the oligoclonal (and occasionally monoclonal) immune response observed in the CNS.
7.3.1.1 Oligoclonal Band Patterns
Table 7.3 shows the five classical patterns of CSF IEF that can be found.
Table 7.3
Oligoclonal band patterns
Type 1: no bands in CSF and serum (S) |
Type 2: oligoclonal IgG bands in CSF, not in the serum, indicative of intrathecal IgG synthesis. |
Type 3: oligoclonal bands in the CSF (like Type 2) and additional identical oligoclonal bands in CSF and serum samples. |
Type 4: identical oligoclonal bands in CSF and serum indicating a systemic rather than an intrathecal immune reaction, with a leaky or normal or abnormal blood–CSF barrier and oligoclonal bands passively transferred into the CSF. |
Type 5: monoclonal bands in the CSF and serum sample seen in the presence of a paraprotein (monoclonal IgG component). |
Type 1 is a negative test result. However, if the clinical picture strongly suggests a diagnosis of MS, then a repeat lumbar puncture may be indicated in any patient with initially OCB-negative CSF. For instance, Fig. 7.3 shows the IEF pattern of a patient with a clinical isolated syndrome (CIS) in 2004 and again 18 months later. Clearly, a Type 2 pattern has developed and the patient then fulfilled the criteria for definite MS.


Fig. 7.3
The CSF in a patient presenting with CIS who showed no evidence of intrathecal IgG in 2004 but developed oligoclonal IgG bands in 2005
Type 2 is also straightforward: specific bands are present in the CSF but not in the serum. This pattern is observed in patients with MS. MS is probably the disease with the strongest stimulation of the B-cell clones within the CNS parenchyma, but any process triggering a B-cell response may lead to the presence of IgG in the CSF. Other diseases known occasionally to produce an oligoclonal IgG response are: paraneoplastic disorders; systemic lupus erythematosus; Bechet’s disease; Sjogren’s syndrome; cerebral angiitis; and CNS infections such as aseptic meningitis, neuroborreliosis, neurosyphilis, HIV infection. An oligoclonal pattern in the CSF is pathological and requires further investigation.
The interpretation of Type 3 and 4 patterns is more complex [31]. In particular, Type 4 can be misinterpreted if the amount of IgG in the serum is too high, as this can blur the serum bands. This is one reason for adding equal amounts of IgG from the CSF and the serum sample [31, 37]. Type 4 can be seen in conditions such as Guillain-Barré syndrome.
Type 5 indicates the presence of a monoclonal gammopathy, but IEF resolves what would be a single band using other electrophoretic techniques, into multiple bands differing by 1 U of charge. This peculiarity is probably due to posttranslational modifications such as glycosylation. McCombe et al. found a CSF monoclonal band in 3/1,490 of CSF samples [38]. The diagnoses were lymphoma or lymphomatoid granulomatosis within or adjacent to the nervous system in two patients and a chronic inflammatory demyelinating polyneuropathy in the third. Ben-Hur et al. (using the less sensitive agarose electrophoresis) described 20 patients with a CSF monoclonal band [39]. The diagnoses were clinically definite MS, probable MS, CIS, SLE, paraneoplastic syndrome, peripheral neuropathies, superficial siderosis, and torsion dystonia.
Davies et al. performed a repeat lumbar puncture in 31 patients who had a monoclonal band in the CSF but not in the serum [40]. All patients who developed clinically definite MS also converted from an intrathecal monoclonal to an oligoclonal pattern.
7.3.1.2 Oligoclonal Band Numbers
The hypothesis behind counting bands is that a higher number of bands may be of prognostic or diagnostic value. For example, a recent paper by Bourahoui et al. concluded that the presence of ≥10 bands in the CSF was highly specific for MS [41]. Two groups found that the absence of OCBs in the CSF of patients with MS is a good prognostic sign [42, 43].
The Queen Square study was based on 12 patients, 6 of whom had a repeat CSF, with 3 becoming OCB-positive and the other 3 remaining negative [42]. This data is in line with another group which suggested that absence or low numbers of bands predict a better prognosis [44]. In contrast, a very recent paper by Koch et al. on 143 patients (110 OCB-positive, 33 OCB-negative) did not find any relationship between the presence and number (or absence) of CSF OCB bands and either disease progression or MS subgroups (relapsing–remitting, secondary progressive, primary progressive disease) [45]. The percentage of OCB-negative MS patients (23%) in this study was higher compared to the studies shown in Table 7.2, with all groups using IEF followed by IgG-specific immunofixation.
However, counting the number of bands in the CSF may not be a true reflection of the number of B-cell clones producing the bands. Posttranslational modification of IgG (see Fig. 7.4) probably also includes changes in immunoglobulin disulfide bonds which changes the pI, resulting in a differential migration in the electric field. Therefore, different IgG bands seen on the immunoblot may originate from the same clone. In order to address the biological relevance of OCBs, the number of clones producing the bands (reflecting the vigor of the immune response) may turn out to be more relevant than merely the number of bands present.


Fig. 7.4
Representative diantennary-type oligosaccharide structure found in association with the Fc moiety of human polyclonal IgG molecules. Straight lines indicate the core and dotted lines the outer arm sugar residues. Differential glycosylation at these residues can change the net charge of the IgG molecule and thus its IEF migration (Reprinted by permission from Macmillan Publishers Ltd: reference [46])
7.3.1.3 Improving the Detection of Immunoglobulins
One B-cell clone can only express either kappa or lambda light chains with kappa being quantitatively the dominant light chain in the human body. As a result, the kappa light chain (free and bound) is found more frequently in the CSF than lambda. Immunoblotting for light chains decreases background (noise) due to the higher antibody specificity which leads to clearer immunoblots. In practice, immunoblotting for kappa/lambda light chains may be helpful in a number of situations. When a “ladder” (Fig. 7.5) is seen with total IgG (which could be due to posttranslational modifications of one single IgG molecule), kappa/lambda staining decides whether the IgG is monoclonal since monoclonal IgG only stains for one light chain. In addition, if there is uncertainty whether very faint bands are present, kappa/lambda staining may increase the sensitivity of detection. In one study [47], we found that the sensitivity for detecting OCBs in CSF in clinically definite MS patients increased from 89% to 98% using kappa/lambda staining (unpublished data). Given this, it may be interesting to reanalyze the CSF of those MS patients reported to be OCB-negative, using kappa/lambda staining [42, 43, 45]. Finally, if there is “negative staining” (looking very white at the beginning of the blot, toward the cathode), this may be due to IgM which is not picked up by the IgG staining and kappa/lambda can be of help.


Fig. 7.5
A “ladder” is observed for total IgG. In this situation, it is useful to stain for kappa and lambda light chains
As in any immune response, IgM levels increase in the serum and CSF before IgG develops. Sensitive and specific detection of CSF oligoclonal IgM bands is possible using IEF [48]. As with IgG, IgM is not specific for MS but is also found in other inflammatory CNS diseases [49]. It has been suggested that oligoclonal CSF IgM is of prognostic relevance in MS [50]. Again, it would be interesting to reanalyze those MS patients without evidence of intrathecal IgG production [42, 43, 45] for intrathecally produced IgM.
7.3.1.4 Antigenic Targets for Intrathecally Produced IgG
In theory, identification of antigens targeted by intrathecally produced IgG could go a long way to revealing the cause(s) of MS. So far, these attempts have not been successful. Part of the problem is that most of the CSF IgG in MS is of low affinity [51–53]. There are, however, situations where high-affinity antibodies are found in the CSF. These are mostly directed against viral antigens. Antigen-specific immunoblotting is a convenient technique for detecting these specific antibodies in a laboratory which is already set up for IEF of OCBs [54]. One example of such an immunoblot is shown in Fig. 7.6.


Fig. 7.6
The antigen-specific immunoblot (lower panel) pulls out monoclonal IgG (the IgG is distributed in a ladder pattern) directed against VZV from the oligoclonal pattern (upper panel)
Typically, patients with subacute sclerosing panencephalitis (SSPE) have CSF IgG directed against the measles virus. In a similar manner, an immunoblot against measles-specific antigens can help to identify them (see Fig. 7.7).


Fig. 7.7
Local synthesis of measles-specific oligoclonal IgG in Subacute Sclerosing Pan Encephalomyelitis (SSPE)
The search continues to identify specific antigenic response in the CSF which may be causally related to MS susceptibility. If the total IgG and antigen-specific IgG patterns share >50% of bands, this would probably indicate a causal relationship. However, the process of intrathecal synthesis of IgG is a dynamic process, making interpretation of (usually) a single diagnostic CSF analysis at disease presentation difficult to fully interpret.
7.4 Cell-Type-Specific Protein Biomarkers in MS
The importance for biomarkers in neuro-axonal degeneration cannot be overestimated. For a patient, loss of function due to axonal loss is likely to be permanent [4]. In simplified terms, loss of function or disability may be caused by demyelination and conduction block, both of which are reversible, and/or axonal loss which is irreversible (Fig. 7.8).


Fig. 7.8
Disintegration of axonal membranes leads to release of axonal proteins into the ECF and CSF as described above (Fig. 7.9). Biomarkers relevant for axonal degeneration in MS research have therefore attracted much interest (Table 7.4).


Fig. 7.9
Biomarkers are released following brain damage. Here axonal damage is taken as an example to illustrate how neurofilaments (Nf) are released into the extracellular fluid (ECF) as the axonal membrane disintegrates. Once released into the ECF, these biomarkers diffuse into the CSF from where they can be quantified following lumbar puncture (Figure reprinted with permission from Nova Science Publishers, reference [16])
Table 7.4
Review of the literature on the relationship of CSF biomarkers with disability in MS patients
Biomarker | Correlation with disability |
---|---|
Neuro-axonal | |
Neurofilaments | |
Protein 14-3-3 | No quantitative data available |
NSE | Not examined |
Astrocytes | |
GFAP | |
Glia | |
S100B | |
Ferritin | No [47] |
Glial and axonal | |
Tau | Possibly [59] |
Axonal biomarkers can distinguish between MS subtypes [56–59]. Of these, neurofilaments have been most consistently found to be of prognostic relevance [56 58–66]. At present, neurofilaments are probably the most promising axonal biomarker in conditions with substantial axonal loss [57, 62, 68–70]. The concept has been validated in animal and cell culture experiments [71–75]. A number of analytical techniques have been developed to this purpose [61, 68, 76–79]. Additionally, the sandwich ELISA has been commercialized (Millipore and BioVendor; Fig. 7.10). For example, analysis of spinal cord tissue from chronic relapsing experimental autoimmune encephalomyelitis (CREAE) homogenate using an ELISA technique shows that the proportion of tissue NfHSMI35 was significantly lower in CREAE animals compared to controls, and was consistent with axonal loss (Fig. 7.10b).




Fig. 7.10
(a) Immunocytochemistry on longitudinal fresh frozen spinal cord sections of three control and three CREAE animals (×40). NfH staining in white matter. Axonal tracts can be followed in control but not in CREAE animals. The staining in CREAE animals is less intense and many axonal end-bulbs are seen. (b) Mice spinal cord tissue homogenates. Scatter and box-whisker plot for NfHSMI35 (ng/mg protein) (Reproduced with permission from Elsevier, reference [71])
Another important advantage is that the phosphorylated neurofilament heavy chain can also be quantified from the blood in patients with ON and MS [60, 66, 77].
7.4.1 Posttranslational Modifications of Biomarkers
A list of cell-type-specific biomarkers was presented in Table 7.1. Many of these biomarkers are proteins and many proteins undergo physiological changes called posttranslational modification (PTM). PTMs change the physical properties of proteins and are processes crucial to a wide range of cellular functions, such as cell signaling and structural stability. Most PTMs are predetermined by the amino acid residues of the protein. For example, the amino acids serine, threonine, and tyrosine are particularly suitable for adding a phosphate ion (phosphorylation). As proteins age and get damaged further, PTMs such as oxidation occur. Most PTMs are targeted to “hotspots” in the protein. For example, oxidation and glycation are typically targeted on amino acid residues exposed at the protein surface, easily accessible to reactive molecules. Analysis of PTMs may be useful in determining a range of pathological processes and may prove useful in detecting subclinical disease activity related to older MS lesions and thus inform on disease progression. For example, in MS there is some evidence that the phosphorylation of heavy chain neurofilament (NfH) is changed in the MS brain [80]. This is consistent with the in vivo finding of increased CSF levels of NfH phosphorylation in patients who reach the secondary progressive phase of their disease [58] and those who are more severely disabled, using the newly developed Global Multiple Sclerosis Severity Score (MSSS) [67].
Phosphorylation is only one of the many posttranslational modifications biomarkers may undergo with disease. Mechanisms of covalent protein modifications comprise co-translational, posttranslational, and spontaneous mechanisms. The mechanisms most relevant to CSF biomarker analysis have been summarized in Table 7.5, and fall principally into two categories: enzyme-mediated, which should only occur in vivo and can be scrutinized for disease-specific signatures; and spontaneous, which will affect proteins in vivo and in vitro and are therefore difficult to study because of artifactual modifications.
Table 7.5
Mechanisms of in vivo and in vitro posttranslational modifications (PTM) relevant for CSF biomarker analysis
Mechanism | In vitro | In vivo |
---|---|---|
Glycosylation | No | Yes |
Phosphorylation and dephosphorylation | No | Yes |
Citrullination | No | Yes |
N-terminal modifications | No | Yes |
C-terminal modifications | No | Yes |
Deamidation | Yes | Yes |
Cross-linking | Yes | Yes |
Oxidation | Yes | Yes |
Nitrosylation | Yes | Yes |
Glycation | Yes | Yes |
Isomerization | Yes | Yes |
Racemization | Yes | Yes |
Proteolysis/cleavage | Yes | Yes |
PTMs of particular relevance to biomarker research include:
Glycosylation: Glycosylation is an enzymatic process that attaches glycosyl groups (saccharides) to proteins, lipids, or other organic molecules. Protein glycosylation is central to a range of immune processes. Altered protein glycosylation may trigger an autoimmune response [81]. Glycosylation may affect charge and susceptibility of the protein to proteolysis.
Phosphorylation and Dephosphorylation: Phosphorylation is essential for intracellular signaling. A range of kinases mediating phosphorylation and dephosphorylation have been described, far exceeding the scope of this review (for selected reviews, see references [82–94]). Proteins can be immunogenic, depending on their phosphorylation status [81, 95].
Citrullination: Citrullination (synonymous: peptidylarginine deimination or just deimination) is the PTM of the amino acid citrulline into arginine. The reaction is catalyzed by peptidyl arginine deaminases in a Ca-dependent manner [96]. Citrullination affects charge and susceptibility to proteolysis.
Deamidation: Deamidation is the removal of an amide group from an organic compound, which affects protein charge and may also affect susceptibility to proteolysis. The reaction is catalyzed by tissue transglutaminase (tTG) in a Ca-dependent manner. A range of autoantigens are substrates to tTG, the most understood being gliadin in celiac disease [97].
Glycation: Glycation leads to the formation of advanced glycation end products (AGEs). The complex reactions leading to AGEs are caused by protein condensation, rearrangement, and fragmentation occurring in concentrated sugar solutions. Many AGEs are unstable and some are immunogenic [81]. Antibodies against AGE-modified low-density lipoprotein (LDL) have been demonstrated in patients with type I diabetes [98]. Protein charge and susceptibility to proteolysis can be changed.
Oxidation: Oxidation changes protein charge and susceptibility to proteolysis. Enzymes involved in oxidative damage are nitric oxide synthethase (NOS), cyclo-oxygenase (COX), mono-amine oxidase B (MAO-B). Spontaneous oxidation occurs with ionizing radiation, reduction of metal ions (Fe(II), Cu(I)) or chemical compounds. Oxidating compounds such as free radicals are commonly known as reactive oxygen species (ROS).Stay updated, free articles. Join our Telegram channel
Full access? Get Clinical Tree
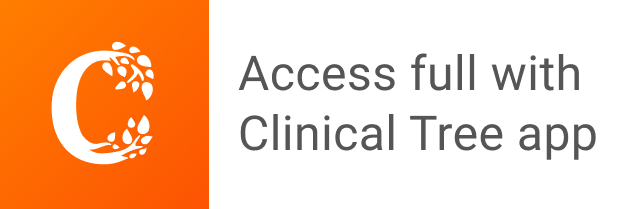