© Springer International Publishing AG 2017
Luigi Manfrè (ed.)Vertebral LesionsNew Procedures in Spinal Interventional Neuroradiologyhttps://doi.org/10.1007/978-3-319-52634-8_22. Biomechanics of Vertebral Fracture
(1)
Orthopaedic Biomechanics Laboratory, Holland Musculoskeletal Program, Sunnybrook Research Institute, 2075 Bayview Avenue, Room S620, Toronto, ON, Canada, M4N3M5
(2)
Department of Surgery, IBBME and IMS, University of Toronto, Toronto, ON, Canada
Keywords
VertebraBiomechanicsStructural stabilitySpine2.1 The Spine as a Biomechanical System
The spine is a complex mechanical system complete with levers, pivots, passive restraints and actuators in the form of vertebrae, intervertebral discs and facet joints, ligaments and muscles, respectively [1, 2]. The bony spine consists of 24 vertebrae (7 cervical, 12 thoracic and 5 lumbar), the sacrum and coccyx. Each vertebra consists of a vertebral body (the primary load-bearing structure) and posterior elements. Vertebrae protect the neural canal and form the primary load-bearing structure within the spine.
The study of spinal biomechanics and injury dates back to the Egyptian empire during the time of the construction of the pyramids [2, 3]. Modern biomechanical studies aim to better understand the mechanics of musculoskeletal motion and the mechanical behaviour of biological tissues and structures including the spine. The importance of biomechanics is present both at structural and tissue levels in physiologic, pathologic and traumatic injury scenarios. Each element of the spine is intricate with respect to its structure, function and its interactions within the system as a whole. Biomechanical analysis is important in understanding vertebral fracture risk, the impact of treatments on the bone and the design and performance of implants and minimally invasive repair techniques for the spine. This chapter will specifically focus on the biomechanics of vertebral fracture in the spine.
2.2 Vertebral Structure
Vertebrae consist of four main structural components: the trabecular centrum, the cortical shell, the bony endplate composing the vertebral body and the posterior elements.
2.2.1 Trabecular Centrum
The trabecular centrum is the principal load-bearing component of the vertebra [4–7]. Trabecular bone density varies with location, spinal level, age, sex and pathology [4, 8–11]. Loss of density is reflected through both trabecular thinning and loss of trabeculae, leading to architectural changes from plates to rods with a sparser network of longer vertical and horizontal struts.
2.2.2 Cortical Shell
The thickness of the cortical shell ranges from 0.3 to 0.4 mm and is thickest near the endplates [12–14]. While the mechanical properties of the cortical shell are reported to be comparable to cortical bone at other anatomic sites [15, 16], its microstructure is more representative of condensed trabecular bone [13, 17, 18]. The cortical shell has been reported to support from 10% to 75% of spinal axial compressive loading [5, 19, 20]. However there is a mechanical interaction between the cortical shell and the centrum [21]. Intervertebral disc degeneration increases the structural role of the cortical shell, and the shell may also support more load at the mid-vertebral transverse cross-section than nearer to the endplates [22].
2.2.3 Endplates
Vertebral endplate thickness ranges from 0.4 to 0.8 mm [12–14]. The morphology of the vertebral endplate is altered with advancing disc degeneration. While early research suggested age-related thickening of the vertebral endplates, more recent microCT-based analysis has shown disc degeneration leads to decreases in endplate thickness and increases in porosity [23–25]. Failure loads and stiffness measurements are consistent with changes in endplate thickness with axial position (level) and across the endplate (thinner central portion). Mechanical testing has shown a significant decrease in endplate stiffness and strength in the vertebrae adjacent to more degenerated discs [26, 27]. Vertebrae may also demonstrate ‘double endplates’, most commonly adjacent to grade 2 or 3 degenerated discs [25, 28]. With the transfer of load through the intervertebral disc, the endplates deform transferring load to the underlying trabecular centrum.
2.2.4 Posterior Elements
The posterior elements consist of two pairs of facet (apophyseal) joints connecting adjacent vertebrae in the inferior and superior directions. The posterior elements play a significant role in torsion, transverse shear and extension loading modes [29]. The orientation of the facets varies with spinal level, enabling a wide range of movement in the cervical spine (flexion, extension, lateral flexion and rotation), limiting flexion/extension movements in the thoracic spine and preventing rotation in the lumbar spine. The posterior ligamentous complex functions as a tension band and protects against hyperflexion. While only a small portion of the compressive load on the spine is carried by posterior elements under physiologic loading (<10%), in cases of disc degeneration, this rises substantially (≥40%) [29, 30].
2.3 Vertebral Bone
2.3.1 Composition
The majority of the vertebral body is composed of trabecular bone (70–80%), which is made up of microstructural rods and plates connected in a 3D network generating an open porous cellular solid [31]. The pores are filled with bone marrow. The orientation of the trabecular microstructure in the vertebra is anisotropic with an inferior–superior principal material directionality. Trabecular bone is highly porous and as a result much less rigid (90–400 MPa) than cortical bone.
The trabecular bone tissue is similar to cortical bone, but is arranged in packets of lamellar bone [32] rather than osteons as in cortical bone. The bone tissue is composed of a mineral phase, 43% by volume (hydroxyapatite); an organic phase, 32% by volume (primarily type I collagen); and interstitial fluid, 25% by volume. The apparent mineral density of human trabecular bone ranges from 0.1 to 1.0 g/cm3. Both density and architecture play an important role in determining the mechanical properties of trabecular bone [33–35]. This may include potential detrimental effects of increased variability in trabecular thickness and number within trabecular bone specimens [36, 37]. Material properties in trabecular bone decrease more rapidly with disease and ageing than in cortical bone because of higher rates of turnover.
2.3.2 Mechanical Behaviour
It is the composite nature of the bone and its intricate structure that creates its remarkable mechanical properties: stiff, strong and tough. The organic phase of the bone governs the plastic behaviour of the material and is responsible for much of the bone’s toughness and ductility. The mineral phase of the bone controls its elastic behaviour and largely determines the bone’s stiffness and strength. This division of mechanical properties between the two phases is evident from experiments whereby one of the constituents is damaged. The bone without collagen (due to protease treatment or ashing) or with damaged collagen (due to KOH treatment, irradiation or heat treatment) retains its stiffness [38–41]; however, it is very brittle, failing at lower strains with little mechanical energy input. The bone’s collagen matrix has better mechanical properties (strength, stiffness, fracture toughness) due to mineralization. Experimentally removing the mineral phase in the bone leads to greatly reduced stiffness, with half of the bone’s toughness retained [42].
Mineralization occurs after osteoid (new bone) is formed, but the degree of mineralization in bone tissue is not constant, with the stiffness of the bone increasing with the presence of more mineral. The organic phase of the bone governs the plastic behaviour of the material influencing its toughness and ductility. The bone exhibits directional differences in both strength and toughness.
The apparent stress–strain behaviour of vertebral trabecular bone exhibits an initial linear region followed by apparent yield and failure. Vertebral trabecular bone is anisotropic in both modulus and strength, although the strength of vertebral trabecular bone is only slightly lower in tension than compression [43]. However, its post-elastic behaviour is quite different.
Bone structures damage with everyday loading; however, damage within the bone does not prevent the bone from performing its mechanical function. Bone damage during the linear phase of loading is likely involved in the regulation of bone turnover. Both the resistance to crack creation and growth are important in understanding bone mechanical failure. Vertebral trabecular bone fractures at apparent strains of approximately 1.5% in tension [44]. Under compressive loading, there is a long post-yield plateau in which fractured trabeculae fill the marrow spaces, after which additional loading yields an increase in modulus [45]. Vertebral trabecular bone is transversely isotropic with substantially higher modulus and strength in the inferior–superior direction (similar to the microstructural orientation). There is a strong linear correlation between the stress at which the bone fails and its elastic modulus, whereas there is little to no dependency of failure strain on density [44]. As such, simple strain-based failure criteria are commonly applied to the bone, independent of orientation, loading or density [14].
Mechanistically, bone fracture has been described to occur through strain-controlled failure, indicating ductile behaviour [46–48]. The mineral component of the bone contributes its strength and stiffness; however, as mineralization increases, bone tissue becomes brittle, reducing the energy required for failure [49–53]. Many studies have demonstrated strong correlations between mineral content and strength/stiffness [49, 51, 52, 54].
Cyclic loading and its associated damage and strain accumulation can weaken vertebrae [55–57]. Cyclic loading tests have indicated failure through a gradual creep-like increase in mean displacement and rapid crack propagation from the cortical shell to the interior of the vertebral body [58]. Microdamage and microfractures commonly seen in human vertebrae likely result from cyclic loading that may be accentuated by occasional overloads and may act as a stimulus to remodelling [14]. The process of cumulative ‘fatigue failure’ of the vertebral body has been investigated in human cadaver spines, with the force required to cause fatigue failure decreasing as the number of loading cycles increases [58–61]. Clinical evidence of fatigue failure is observed in the presence of individual fractured and healing trabeculae in many cadaveric vertebral bodies [62].
The compressive strength of human thoracolumbar vertebrae ranges from 2 to 8 kN, based on bone density and cross-sectional area [14, 59, 63–68]. Vertebral compressive strength increases caudally mainly due to increases in vertebral cross-sectional area [68, 69]. Regional distribution of bone density and vertebral geometry also impact strength [5, 8, 59, 63, 68].
2.3.3 Bone Quality
From a biomechanical perspective, the impact of bone quality on fracture risk has gained much recent attention. Where BMD describes only how much bone is present, bone quality describes the mechanical properties encompassing both the inherent structural and material properties within a vertebra. As such bone quality evaluation considers mineralization, composition, remodelling, connectivity and architecture. Together these structural and material factors can be used to determine the load-bearing capacity of a vertebra (failure load) and can reflect the impact of pathological processes (i.e. osteoporosis, diabetes, metastatic disease) or treatments (i.e. bisphosphonates, radiation) on vertebral stability. However, many of these factors are difficult and impractical to measure clinically because of the invasive (bone biopsy) or harmful (high-dose radiation) tests needed to make measurements.
2.3.4 Age-Related Changes
Vertebral body strength decreases by ~12% per decade [67]. The bone is highly affected by ageing with a loss of bone mass of up to 50% from 20 to 80 years of age, influenced by gender, hormonal changes and anatomic site, with negative consequences to its ultimate strength. Architectural changes in the trabecular centrum of vertebrae occur with age, both through thinning and loss of trabeculae. Increase in bone fragility may occur from replacement of plate-like closed trabecular structures with more open structures composed of rods. The more porous trabecular bone appearance results due to reduced horizontal cross-linking struts, further diminishing the buckling strength of vertically oriented (longer and thinner) trabeculae [69]. Reducing bone density by 10% through the removal of longitudinally oriented trabecular elements has been shown to create a 50% greater reduction in bone strength than an equivalent density reduction via trabecular thickness [70]. This implies that maintenance of trabecular number is critical in the preservation of bone strength with ageing [71]. The loss of bone density may be offset in part by subtle increases in bone size in men; however, no age-related changes in cross-sectional area have been found for women [6].
Trabecular microarchitecture has been shown to be highly associated with whole-vertebral biomechanical behaviour, mediated by bone mineral content but not by cross-sectional area or the cortical shell [72]. The role of trabecular microarchitecture is more accentuated in low-strength vertebrae and involves mostly the degree of anisotropy, with variations in vertebral strength primarily due to the bone volume fraction of vertically oriented trabeculae [72]. Localized bending of slender vertical trabeculae has been shown to lead to concentrated yielding at a tissue level in low-density vertebral bone.
Ageing is accompanied by osteoarthritic changes around the intervertebral disc and endplates (i.e. osteophytes) and disc degeneration [73, 74]. Bone density distribution is impacted as well, with more uniform distributions in vertebrae adjacent to degenerated discs [9].
Damage and remodelling of trabeculae are normal physiologic processes that can elevate osteoporotic fracture risk [55, 75]. Decreased structural strength in aged bone is affected by changes in the remodelling/repair rate, resulting in faster damage accumulation for continuous cyclic loading [76]. Ageing slows remodelling, and during remodelling cycles, there is a net loss in bone mass leading to deterioration in crack-initiation and crack-growth toughness. Lower turnover leads to higher mineralization that may contribute to the brittle nature of aged bone tissue.
It is also proposed that elderly vertebrae, with low bone mineral density (BMD), may deform gradually under constant load by a quasi-continuous ‘creep’ mechanism [61, 77]. The bone is a ‘viscoelastic’ material, with repeated loading of small bone samples demonstrating residual deformation that recovers slowly, if at all [78–80]. Since BMD and trabecular density tend to be lower in the anterior vertebral body, creep may contribute in osteoporotic vertebrae to anterior wedge deformities [61].
2.3.5 Impact of Intervertebral Disc
Vertebral body endplate stresses depend on the state of the adjacent intervertebral discs. A healthy disc is composed of a gelatinous nucleus pulposus surrounded by a fibre-reinforced annulus fibrosis. Degenerated discs present with a loss in hydration and height with a nucleus similar to the annulus fibrosis material [81]. Healthy discs preferentially load the interior of the trabecular centrum in axial compression; degenerated discs load the cortical shell in axial compression with an increased load on the anterior body under bending [82, 83]. As such, healthy spinal motion segments tend to fail via central endplate ruptures, whereas low-density vertebrae with degenerated discs fail more commonly in wedge-type fracture patterns [84].
2.4 Spinal Column Classification
Spinal column classifications have been developed to assist in describing fractures from an anatomical perspective. The spine has been described as a two-column structure [85, 86] and a three-column structure [87, 88, 89]. Where the two-column theory divides the vertebrae to the vertebral body and the posterior elements, the three-column concept introduces a middle column, containing the dorsal half of the vertebral body, posterior longitudinal ligament, and dorsal annulus fibrosus. The middle column allows for the specific assessment of the neutral axis, which bears a significant portion of the axial load and about which spinal element distraction or compression does not excessively occur with flexion or extension [90]. The instantaneous axis of rotation (IAR) is generally located within the neutral axis. The posterior column is the most important column for spinal stability, and compromise of any two columns that include the posterior column is generally mechanically unstable.
2.5 Spinal Stability
All joints in the human body are defined by an inherent stability. In the spine, stability relies on the intervertebral disc and ligamentous structures to provide passive restraint and active stabilization from the surrounding musculature. In a healthy spine, the osseous anatomy provides little intrinsic stability, beyond constraining the limits of motion through the facet joints. Instability of the spine is difficult both to define and quantify [91]. Mechanical stability may be defined as the ability of the spine to bear physiological loads, whereas clinical stability encompasses both mechanical instability and the associated pain or neurological damage. Historically, White and Panjabi defined clinical instability of the spine as changes in the patterns of motion under physiological loads which may result in neurologic deficit, excessive deformity and/or pain, acutely or with time [57]. They further described kinematic instability as excessive change in physiologic motion, axis of rotation or in the coupling characteristic of the spine.
2.6 Vertebral Fractures
Spinal fractures can vary widely with respect to both severity and injury pattern and occur more commonly in the thoracic and lumbar spine, including the thoracolumbar junction. Fractures may occur traumatically due to high-impact loading (including motor vehicle accidents, falls from heights and sport-related collisions) or under physiologic loading levels when inherent weakening of the vertebrae is present due to pathologic conditions (such as osteoporosis or metastatic disease).
2.7 Traumatic Injuries
Traumatic spinal injuries cover a large spectrum, including minor sprains and strains, herniated discs (tears in the annulus causing leakage), subluxations, fractures and dislocations of the facet joint [92–95]. In general, spinal trauma is the result of high-speed injuries and is most common among the younger male population [93]. Damage to the spinal cord is present in only a small percentage of these injuries [96, 97].
Early spinal trauma classification systems focused on anatomical, morphological and mechanistic criteria of the trauma [95]. Sir Francis Holdsworth described 1000 patients with facet fractures and dislocations in a widely referenced historical classification system [85]. More complex classification systems have since been developed; however, none is considered ideal [92, 94]. For example, the Allen-Ferguson system divides traumatic injuries of the cervical spine into six phylogenies, compressive flexion, vertical compression, distractive flexion, compressive extension, distractive extension, and lateral flexion, and has been shown to be an effective diagnostic tool [92, 98]. The newer subaxial injury classification (SLIC) system is also largely based around mechanisms of injury but provides evidence on the morphology of fractures, assessment of the discoligamentous complex and neurologic status [94]. The international group AOSpine has provided updated classification guidelines on cervical spine injuries based on common presentations [99]. Soft tissue injuries to the spine can result from hyperflexion which typically results in posterior injuries whereas hyperextension causes anterior ligamentous and disc disruption. Flexion distraction injuries have a spectrum of injury based on facet joint distraction, ranging from subluxation and fracture to dislocation injuries (which have a high propensity to include spinal cord damage). Incidence of these injuries has been shown to result from the location of the force vector applied, shifting from posterior element fracture to bilateral facet dislocation. Additional classification systems exist for the axis and odontoid.
For thoracolumbar injuries, Denis classified five different types of spinal fractures considering the anatomic site of injury, the mechanism of injury and the level at which the injuries tend to occur [88]. The McAfee and Magerl classifications for thoracolumbar spinal fractures are similarly based on the three-column concept of the spine (anterior, middle and posterior), with instability predicted depending on the columnar disruption [100, 101]. More recently, the thoracolumbar injury classification and severity score was developed based on injury morphology (compression fracture, burst fracture, translational rotational injury or distraction injury), posterior ligamentous complex integrity (intact to injured) and patient neurology (intact to cauda equina) [102]. These classifications are designed to help guide operative vs. non-operative management of patients [103].
2.7.1 Osteoporotic Fractures
Vertebral fractures are the most common type of osteoporotic fracture. They are associated with increased morbidity and mortality (20% increase in 5 years) [104]. The prevalence of vertebral compression fracture increases the subsequent risk of fracture in both the spine and hip [105–107]. The high rate of subsequent vertebral fracture following an initial fracture is referred to as the ‘vertebral fracture cascade’ [108]. Only one third of osteoporotic vertebral fractures come to medical attention. Moderate trauma is associated with many painful vertebral fractures, but studies have reported from 50% to 73% as occurring ‘spontaneously’ without any known cause or due to only low-energy trauma [109, 110].
2.7.2 Pathologic Fractures
The vertebral column is the most frequent site of metastatic involvement of the skeleton, occurring in up to one third of all cancer patients [111]. Bone metastases contribute to both morbidity (pain and skeletal-related events) and mortality [112, 113]. Pain management and the prevention of pathologic spinal fractures are critical elements in the preservation of quality of life in individuals with vertebral metastases. Vertebral metastatic disease uncouples physiologic osteoclastic and osteoblastic cell interactions leading to abnormal bone turnover, impacting architecture, density, bone quality and stability [114, 115]. Vertebral metastases can be present as bone destructive (osteolytic), as bone generating (osteoblastic) or as a mixture of osteolytic and osteoblastic disease. In some cancer types (i.e. breast), the incidence of pure osteolytic metastases has declined, likely at least in part, due to the widespread use of bisphosphonates in patients with skeletal disease [116]. Pathologic sequelae can be found in the vertebrae as distinct focal regions and areas with more diffuse involvement throughout entire vertebrae. The time-dependent risk of skeletal-related events, including fracture, is increased with metastatic involvement [117, 118]. Fracture can occur in the metastatic spine under physiologic loading conditions resulting in wedge or burst fracture patterns.
2.8 Fracture Types
2.8.1 Compression Fractures
Compression fractures can present with an anterior wedge, biconcave or crush morphology. Cadaveric studies have shown that some degree of endplate disruption occurs in all types of vertebral compression fracture, suggesting its role as a ‘weak link’ in the spine [10, 119–122]. Wedge fractures, characterized by failure of the anterior wall of the vertebral body, are the most common type of thoracolumbar spinal fracture (~50%). Such fractures generally result from axial compressive loading which may be combined with forward bending moments. Compressive forces applied ventral to the IAR often result in wedge compression fractures [90]. They are generally considered a single-column injury and as such are deemed to be relatively stable. They are largely unreported but can present with pain and loss of mobility. They can be classified as mild, moderate or severe depending on the loss of vertebral height. The most common pathology leading to wedge fractures is osteoporosis, but such injuries can also result from metastatic disease and secondary to trauma. In wedge fractures, an underlying cause of anterior weakening may be ‘stress shielding’ of the anterior vertebral body by the neural arch, following intervertebral disc narrowing [123]. In this scenario, even moderate flexion can disengage the neural arches, concentrating over 50% of the applied compressive force on to the weakened anterior vertebral body. Bone loss in the anterior vertebral body may also result due to limited bending in the elderly coupled with reduced responsiveness to mechanical stimuli of old compared with younger bone [61, 124, 125].
Initial failure of the vertebra is often associated with the development of high tensile strains within the endplate, which in turn is influenced by the material behaviour of the disc [72]. Biaxial tension generated in the endplate may be particularly harmful with respect to limiting microdamage and crack propagation [72]. Biconcave fractures result from both endplates bulging into the vertebral bodies with increasing age [126, 127]. This may occur in middle age, when only the central nucleus pulposus exhibits a true hydrostatic pressure [128], which acts on the weak central region of the vertebral endplates leading to the central region of the endplates bulging into their vertebral bodies when the spine is compressed [10, 121, 122, 129]. Both disc degeneration and vertebral endplate damage can cause a reduction in pressure in the nucleus pulposus of the adjacent disc [130], concentrating compressive stress on the annulus and neural arch. If moderate spinal flexion disengages the apophyseal joints [131], then compressive overload can result in a crush fracture that primarily involves the vertebral body cortex [61].
2.8.2 Burst Fractures
Burst fractures have a similar appearance to crush fractures but with additional radial outward displacement of the cortex. Burst fractures generally occur from high-impact loading in young healthy spines. Pure axial loading in line with the IAR leads to a burst fracture pattern. The mechanism of burst fracture has been described as a pressurization of the vertebral body under rapid loading due to failure of the endplates and entry of the nucleus pulposus into the vertebral trabecular centrum [85]. This pressurization leads to radial bursting of the vertebral body, which can cause pieces of bone to retropulse into the spinal canal causing neurologic damage. This mechanism can also occur in cancer-involved bone under physiologic loading conditions due to pressurization of osteolytic tumour tissue leading to the retropulsion of the bone and/or tumour into the spinal canal [132].
2.8.3 Flexion Distraction Injuries
Distraction forces placed dorsal to the IAR can generate ligamentous or bony ‘Chance’ fractures [90]. The magnitude of the applied bending moment (related to the perpendicular distance of the force relative to the IAR) impacts the extent of the fracture. The most common cause of this injury pattern is deceleration with restraint by a single lap belt. Hyperextension-shear injuries result from compressive forces behind the IAR [90]. Flexion distraction-type injuries have been classified into four stages, based on the severity of post-injury translational displacement [92]. The stages range from (1) representing an isolated posterior ligamentous injury resulting in facet subluxation only in association with post-traumatic flexion to (2) a unilateral facet injury and ultimately gross instability with (3) and (4) which include bilateral facet dislocation/subluxation. Each stage may exhibit a variety of injury patterns including facet fractures, facet subluxation/dislocation (pure ligamentous injury) and vertebral body fractures. The more recent SLIC adds some additional consideration to this injury pattern (considered hyperflexion) for facet subluxations and perched facets [94]. However, the combination of fractures and ligamentous injury that can produce the various stages of injury and the resulting instability pattern remains poorly understood [133].
2.8.4 Facet Joint Injuries
Traumatic facet joint injuries can result in a spectrum of soft and bony tissue disruption [134]. As a primary stabilizer for axial rotation of the head and neck, facet joint injuries are the results of high-energy injuries with flexion rotation moments (unilateral facet injury) and hyperflexion/distraction (bilateral facet injury) [134, 135]. Facet subluxation describes joint motion extending beyond its physiologic range of motion. Excessive facet subluxation can result in facet fractures that can occur in the inferior articular process of the superior vertebrae or in the superior articular process of the inferior vertebrae (a more common occurrence) [93]. Facet perch describes subluxation immediatly prior to dislocation where the ends of the joint are atop one another [94]. Facet dislocation, which can occur unilaterally or bilaterally, is when the joint surfaces have slid past each other and are in a locked position.
2.8.5 Fractures of the Odontoid Process
The most frequent cervical spine injury in the elderly is a fracture of the odontoid process, often referred to as a ‘dens’ fracture, of the second cervical vertebra (C2) [136]. The odontoid process is a unique bony prominence or peg of the C2 vertebrae about which the C1 vertebra rotates. In the elderly population, this fracture type is common in low-energy impacts that occur during a fall from a standing height as a result of weakened bone due to osteoporosis and change in force vectors due to osteoarthritis [136, 137]. The low-energy mechanism of this fracture typically leaves the surrounding ligamentous complex intact which gives support that these fractures are inherently stable [138]. However increased mobility of the C1/C2 complex can result in spinal cord injury and fusion may be required.
2.8.6 Classification of Fracture Instability
Many classification schemes of spinal fracture instability exist, but in general, instability is referred to as acute (overt or limited) or chronic [90]. Acute instability is most frequently encountered in traumatic, infectious, and/or neoplastic conditions. Chronic instability may result from an acute process or degenerative changes. Classification systems for fracture instability aim to determine which fractures are overtly unstable (require surgical stabilization), which fractures have limited instability but with a likelihood of chronic progression (require surgical stabilization) and which fractures are grossly stable (do not require surgical intervention). Overt instability is defined as the inability of the spine to support the torso during normal activity and requires loss of integrity of the vertebral body or disc and the posterior elements. Limited instability refers to the loss of either ventral or dorsal spinal integrity (not both) with overall stability sufficient to support most normal activities. The most widely described mechanistic scheme is the AO classification [100]. Based on the two-column theory, the AO system describes fractures as Type A (ventral column injuries resulting from axial loading with or without flexion), Type B (ventral and dorsal column injury from flexion or extension, with distraction) and Type C (ventral and dorsal column injury from rotational forces). This classification scheme is extensive with subgroups and specifications providing categories for the majority of spine fractures.
2.9 Fracture Risk Prediction
2.9.1 Bone Mineral Density
Bone mineral density (BMD) estimation based on dual-energy X-ray absorptiometry (DXA) remains the clinical standard of care used to estimate osteoporotic fracture risk in the spine. DXA is a 2D imaging technique that yields an ‘areal’ measure of BMD (units g/cm2) obtained by dividing the bone mineral content by the area of a standardized region of interest. DXA measurements alone explain only ~60% of the variation in vertebral strength [63]. Vertebral fractures occur in individuals with BMD values outside the range of osteoporosis in up to 50% of all cases [139]. Correlations between compressive strength of cadaveric vertebrae and BMD are modest (r 2 from 0.62 to 0.69).
2.9.2 Clinical Factors
Fracture risk prediction tools have been developed which rely both on BMD and validated clinical risk factors, such as FRAX developed by the World Health Organization [140]. The FRAX® algorithms give a 10-year probability of hip fracture and overall osteoporotic fracture (spine, forearm, hip or shoulder) based on hip BMD and clinical risk factors (age, sex, height, weight, previous fracture, smoking, alcohol, glucocorticoids and rheumatoid arthritis). In particular, a history of a fragility fracture (vertebral or hip) is a powerful predictor of future fracture independent of BMD and is considered in such risk analysis tools [105, 141]. However, the added benefit of such tools beyond BMD alone for the assessment of vertebral fracture risk has not been conclusive in the literature [141, 142].
2.9.3 Quantitative Computed Tomography
Quantitative computed tomography (QCT) is a 3D X-ray-based method of bone density assessment that provides a true measure of volumetric bone mineral density (units g/cm3). QCT can isolate measurements to the trabecular centrum and yield measures of vertebral geometry, such as cross-sectional area and total volume. However, QCT versus compressive strength of cadaveric vertebrae have yielded only weak to moderate correlations (r 2 from 0.23 to 0.66) with some improvement when both density and cross-sectional area are considered [63, 65, 69]. Neither DXA nor QCT measures of BMD are strong predictors of whether an individual will suffer an osteoporotic vertebral fracture in vivo [143].
2.9.4 Structural Rigidity
Structural rigidity is a measure based upon engineering beam theory that integrates both the material and geometric properties of an object, defining its ability to resist axial, bending and twisting loads via axial (EA), bending (EI) and torsional (GJ) rigidities, respectively. On the basis of the principle that the weakest section dictates the load capacity for a structure, algorithms have been developed and implemented to calculate the minimal rigidity of the bones [144, 145]. CT-based structural rigidity analysis (CTRA) uses serial, transaxial CT images to measure tissue mineral density and cross-sectional geometry and calculates EA, EI and GJ by summing up the modulus-weighted area of each pixel within the bone contour by the position of the pixel relative to the centroid of the bone cross-section [144]. Based on composite beam theory, CTRA does not, however, predict the exact failure load or pinpoint the exact location of an impending fracture but rather seeks to determine a fracture risk threshold for whole bones using simple and reproducible measures. CTRA has been correlated to failure load (R 2 = 0.69) in cadaveric vertebrae with simulated metastatic defects [145] and with yield load (R 2 = 0.89 to 0.62) in rat vertebrae with osteolytic and mixed osteolytic/osteoblastic metastases [146]. However, no relationship was found for healthy rat vertebrae, and the structural rigidity predicted yield load was considerably higher than the experimental yield load in all groups. In a prospective study of vertebral metastatic breast cancer, CTRA demonstrated 100% sensitivity with specificity from 44% to 70% [147].
2.9.5 Finite Element Analysis
Finite element analysis (FEA) is a numerical technique that is used to find approximate solutions to boundary value problems for partial differential equations. In FEA, a large or complex problem is divided into smaller simpler parts (finite elements) which can be modelled with simple equations. The finite elements are then assembled into a larger system of equations that represents the whole problem. FEA allows parametric representation of complex geometric and material property distributions which occur in musculoskeletal modelling and are difficult to represent with other analytical or experimental techniques. FE models can also be parametrically analyzed to investigate changes in loading conditions, geometries, material property definitions/distributions or pathologies. FEA has been successful in predicting failure loads and fracture patterns for bone structures [148, 149]. FEA based on idealized geometries has been used to generate guidelines for vertebral fracture risk prediction in healthy and pathologic scenarios [150].
QCT-based FEA has been proposed as an improved method for estimating fracture risk in the spine [151]. QCT-based FEA has been shown to be highly correlated with ex vivo compressive stiffness and strength [152–157]. It has been shown to perform better than QCT BMD with and without transverse plane cross-sectional area with respect to ex vivo vertebral compressive strength evaluation (r 2 = 0.86) and is associated with fracture even after adjusting for BMD [153, 157, 158]. QCT-based FEA of lumbar vertebrae has also been shown to better discriminate between osteoporotic and non-osteoporotic individuals and fracture and non-fracture cohorts than BMD alone [156, 157, 159]. However, less encouraging results have been found under anterior flexion loading and in predictions of failure patterns and post-yield behaviour [160–162]. The highly non-uniform loading applied through the intervertebral discs and facets may impact these predictions [163–167]. As well, while QCT yields information on the architecture and mineralization of bone structures, it does not provide information on the organic components of the bone, which impacts its plastic behaviour, nor on the fracture resistance of bone tissue.
2.9.6 Damage
The bone is full of small defects and cracks, and the interaction of these cracks within its microstructure influences how both trabecular and cortical bone fail, leading to whole bone fracture. Fracture risk can be increased by the presence of damage by two mechanisms: (1) damage leading to a reduction in material properties locally leading to catastrophic failure or (2) damage leading to systemic changes that decrease bone mass ultimately translating into less structurally sound bone structures.
Under tensile loading, cracks can open, limiting strength and leading to catastrophic failure. In contrast, compression loads may cause cracks to close or compaction of failed bone tissue allowing maintenance of load-bearing capacity. Both the mineral and organic phases contribute to fracture resistance, through multiple mechanisms at multiple-scale levels, including crack deflection, uncracked ligament bridging, collagen fibril bridging, microcracking, sacrificial bonds, fibrillar sliding and molecular uncoiling [168]. Microdamage in age-related fractures may be due to repetitive loading of the bone, likely initiating at the level of the collagen fibre or below and may include collagen fibre–matrix debonding, disruption of the mineral–collagen aggregate and failure of the collagen fibre itself [55].
Overloading of trabecular bone causes damage that leads to large reductions in apparent modulus [169]. In vertebral specimens loaded in compression to 15% strain, the primary mechanism of failure was found to be microscopic cracking as opposed to fracture of individual trabeculae [170]. Fracture of trabeculae is primarily in elements oriented transversely to the loading direction. Microdamage, rather than microfracture, is likely the mechanism for the large apparent reductions in mechanical properties after compressive overloading [171]. The impact of microdamage on bone strength supports the elevated risk for vertebral osteoporotic fractures in those who have previously sustained osteoporotic fractures. Further, musculoskeletal injury has been shown to lead to a loss in systemic bone mass and specifically decreased stiffness in trabecular bone [108].
Studies have evaluated the impact of age and bone diseases on cross-linking [172]. Interfacial bonding between the mineral and organic phases of bone tissue may also be important to the strength and stiffness of the bone; however, the nature of collagen–mineral interactions in the bone remains less characterized [173–175]. The biomechanical impact of collagen in the bone has been shown to be related to the content present, its extent of cross-linking (interfibrillar pyrrole or intrafibrillar pyridinoline) and gene polymorphisms [176–181]. Collagen cross-linking has also been shown to affect the mineralization process and influence microdamage formation [172]. Fracture energy is highly affected by collagen fibre orientation. The angle between collagen and crack propagation direction influences toughening mechanisms [48]. Woven bone, consisting of unorganized collagen fibrils, shows reduced mechanical properties versus lamellar bone, despite mineralization, highlighting the importance of collagen fibre orientation [182].
The mechanical properties of the bone’s collagen fibril matrix can be influenced by inter- and intrafibrillar cross-links. These cross-links can be formed enzymatically through the action of lysyl oxidase or non-enzymatically through glycation or potentially oxidative stress [111, 183]. Whereas the presence of enzymatic cross-links has been positively correlated to mechanical performance with minimal content changes throughout adulthood [176, 184], increased non-enzymatic cross-linking in the collagen matrix has been shown to degrade the mechanical properties of the bone. Glycation, both in vivo (Advanced Glycation End Products) and ex vivo (ribosylation), changes how the collagen matrix can deform and alter apparent yield strain, strength, as well as changing how microdamage accumulates without affecting the initial stiffness [185–188]. Fragility of ribose-treated bone has been shown to be reversible with a sugar cross-links cleaver (N-phenacylthiazolium bromide) [189]. It has been further verified that increased cross-linking between collagen fibres lowers toughness [189]. High densities of these resulting advanced glycation end products (AGEs) increase trabecular bone fragility, decreasing post-yield strain energy absorption and specifically decrease the energy dissipation within the organic phase [187]. Pentosidine, an AGE-based non-enzymatic cross-link, has been correlated with the strength of individual trabeculae [190]. Pentosidine levels have been shown to increase significantly with age and pathological conditions making it a potential biomarker for bone quality [191, 192].
2.9.7 Loading
The loading applied to the spine is also a critical component of vertebral fracture risk prediction. Both the magnitude and direction of the applied loads are key factors in fracture risk evaluation and in the mechanism of failure. This includes traumatic loading and repetitive loading at physiologic load levels (fatigue failure) [10, 60, 193]. Estimated forces applied to the thoracolumbar spine in older individuals range from approximately 400 to 2100 N for typical activities [71]. Subtle changes in body position can dramatically alter spinal loading. Lifting while standing straight with outstretched arms in comparison to a position with knees bent and arms in greatly increases spinal loads. Forward flexion further increases compressive loading. Activities of daily living such as bending, lifting and rising from a seated position can generate high forces on the spine [71].
A factor of risk can be determined by comparing the load-bearing requirement of the spine to its load-bearing capacity. The ratio of the load on the spine to the failure load of the bone indicates whether fracture is likely during a given activity [193]. A high factor of risk can result from weakened bone (low failure load) or the application of large forces. The likelihood for nonphysiologic spinal loading may be increased through work- or sport-related exposure or pathological processes which may increase the propensity for falling. The relationship between fall risk and skeletal fracture has received much attention in the context of osteoporosis. Muscle weakness, impaired balance and slower reaction times in fall prevention have been suggested as contributors to osteoporotic fracture incidence [108, 193].
2.9.8 Spinal Alignment
While a straight spine would theoretically be an ideal axial loading spinal configuration, it would not tolerate eccentric loads and would provide limited flexibility [90]. The spine has a curvilinear sagittal conformation with a primary kyphotic thoracic curve, compensated by secondary cervical and lumbar lordotic curves of equal summative magnitude, yielding a balanced configuration necessary for a bipedal upright posture [90]. Increases in thoracic kyphosis or the loss of lumbar lordosis results in an increased moment arm generating greater bending moments at each vertebral segment [90]. As such, increased deformity resulting from vertebral fracture can increase the moment arm length, leading to higher bending moments and increased risk for subsequent fracture.
2.10 Vertebral Metastases
2.10.1 Strength and Fracture Risk
Many methods have been employed towards assessing metastatically involved vertebral strength including clinical, experimental, image-based and computational analyses [132, 145, 150, 194–208]. Clinically, preoperative condition influences outcome in the metastatic spine [195]. Qualitative (radiographic-based), experimental and computational modelling of osteolytic vertebrae have found tumour size to be the most important risk factor for vertebral body failure, yet measures of tumour size alone have not been able to completely explain the strength reductions [145, 150, 194, 196, 197, 199, 200, 203].
The presence of contained tumour tissue causes an increased propensity for vertebrae to pressurize and burst into the spinal canal [132]. Osteolytic tumour tissue consists of both solid extracellular matrix and interstitial fluid and can be modelled as a poroelastic material [209]. Vertebral pressurization has been shown at physiologic load levels in specimens with simulated metastases, causing the development of increased tensile hoop strains and tensile axial strains on the vertebral cortex, leading to narrowing of the spinal canal [194]. Digital image correlation under axial compressive loading has shown that higher strains result in the presence of osteolytic tumour, particularly adjacent to the dorsal wall which may correspond to an increased burst fracture risk [204]. At high loading rates, the presence of a large fluid-rich relatively incompressible osteolytic tumour can provide axial support to the vertebral body while increasing pressure, vertebral bulge and tensile hoop strain (indicative of an elevated risk of burst fracture prior to endplate failure). Validated 3D poroelastic FEA of the metastatic spine has demonstrated that while the strongest (exponential) factor is tumour size, lower BMD, pedicle disruption and focal osteolytic disease (in contrast to more diffuse lesions) in the posterior vertebral body also increase burst fracture risk [194, 203, 206]. Additional structural and geometric factors including upper thoracic vertebral level, a reduced degree of kyphosis, removing the stabilization of the rib cage and axial compressive loading lead to a higher risk of burst fracture initiation [206–208].
Experimental in vitro testing has shown metastatic compromise of the posterior elements to cause decreases in vertebral strength, whereas the impact of defect location has been less clear [37, 197]. However, the majority of the mechanical testing protocols have modelled defects as voids or with unconstrained simulated tissue, reducing any potential effects due to the mass and fluid behaviour of tumour material and the ability to study burst fracture patterns [145, 196–200, 202].
Minimum failure load calculated based on axial and bending rigidity has been successful in predicting fracture prospectively in cadaveric studies of vertebrae with simulated and actual lesions and in retrospective evaluation of clinical imaging data [147, 201]. This approach has been shown to be highly sensitive and moderately specific to vertebral fracture [147]. FEA has also been used to develop biomechanically-based guideline equations to quantify burst fracture risk in metastatically involved vertebrae based on load-bearing capacity and load exposure [150, 194]. The equation-based guidelines were able to describe the mechanical behaviour of the metastatically involved vertebral FE model (R 2 = 0.97) reflecting the in vitro risk and mechanism of fracture and yielding a clear clinical threshold for burst fracture risk in a retrospective clinical data set [210].
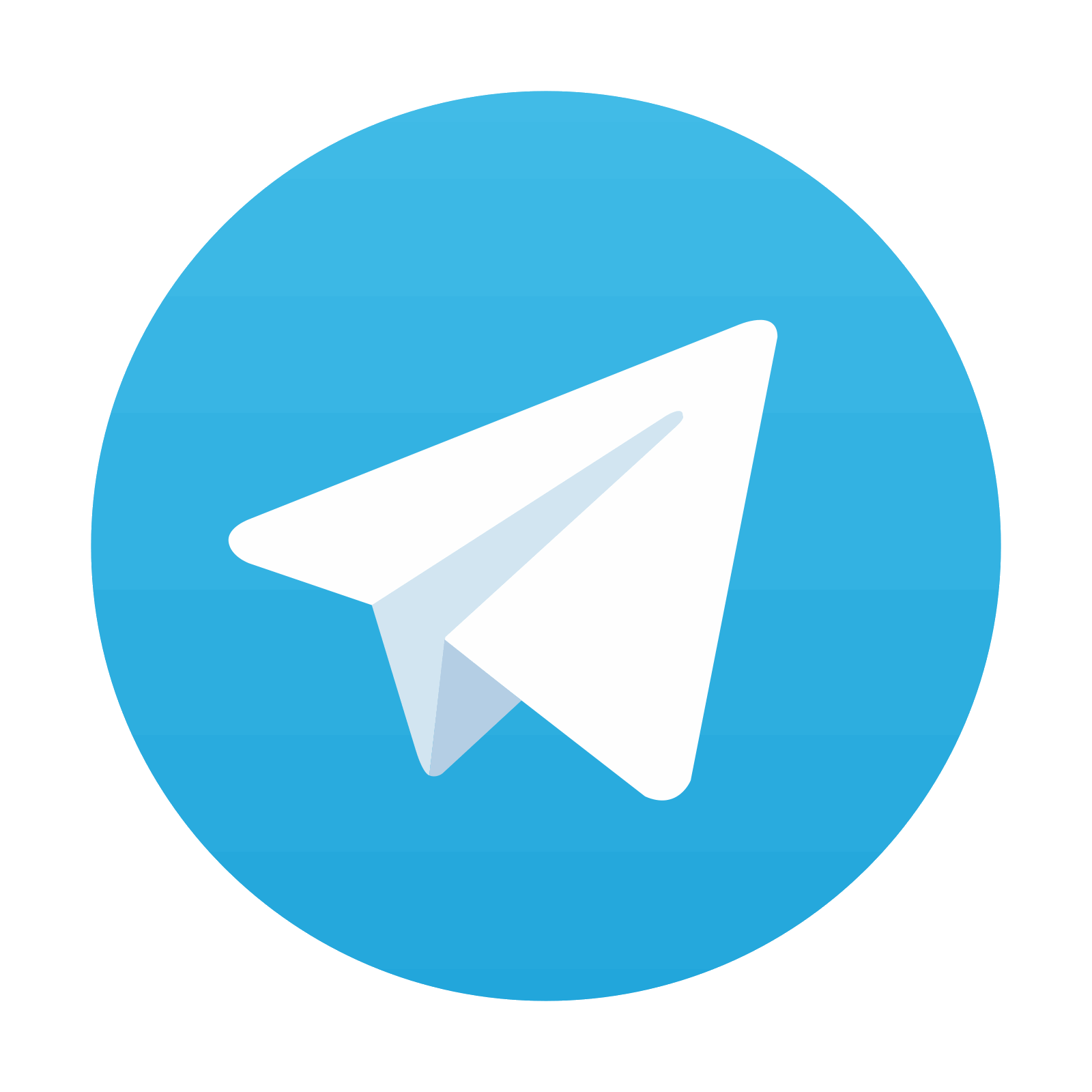
Stay updated, free articles. Join our Telegram channel
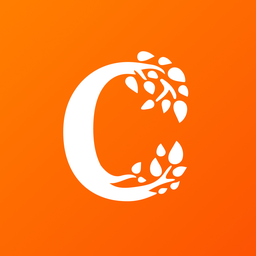
Full access? Get Clinical Tree
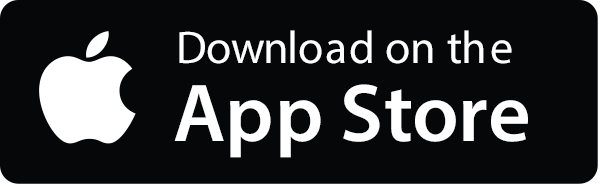
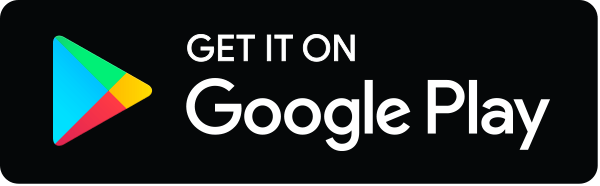