Nonhuman primate
Rat
Mouse
Morphine
IV 0.5, 2.5 mg/kg [13]
IV 0.2–2 mg/kg PR [19]
IV, dose unknown [24]
IV 0.1 mg/kg [14]
IV: 0.1 mg/kg/infusion [25]
PO
IV 0.25 mg/kg/inj [15]
Oral (bottle choice) 0.25–0.5 mg/mL [68]
IV(0.125–4.0 mg/mL) [23]
Fentanyl
Oral 50–75 μg/mL, FR4, FR6, PR [21]
Oral (bottle choice): 25 μg/mL [68]
Oxycodone
SC (0.20 mg/kg) [99]
IV (0.1 mg/kg/infusion) [98]
(0.0003–0.3 mg/kg/infusion) [99]
Hydrocodone
SC (0.032–10 mg/kg. Note: 32.00 mg/kg lethal dose) [112]
IV (0.02–32 mg/kg/infusion) [113]
_
Hydromorphone
IV (1 mg/kg/day) [136]
IV (10–100 μg/kg) [45]
_
Methadone
Oral (low to high- 0.05, 0.2, 0.8 mg/mL given in 0.65 mL volumes) [161]
_
IV (0.03–0.25 mg/kg/inj) [162]
IV (0.179–11.86 mg/kg/day) [137]
Tramadol
IV (0.1, 1.0 mg/kg/inj) [173]
Oral (32–56 mg/kg, FR10) [174]
_
IP (10 mg/kg) [171]
Remifentanil
IV (0.25–32 μg/kg) [190]
_
(10–0.10 μg/kg/infusion FR1) [191]
IV (0.0001 mg/kg/infusion, FR 30) [196]
(0.4, 0.8, 1.6, 3.2, or 6.4 mg/kg/infusion, FR1) [192]
IV 0.025–0.8 μg/kg/injection, PR) [195]
IV (0.25, 0.5, 1, 2, 4, 8, 16, and 32 μg/kg per infusion, PR) [194]
IV: 0.09–2.9 μg/kg/inj [197]
Blood–Brain Barrier Transport
An important aspect of drug distribution involves a set of proteins positioned within the endothelial cells of the CNS vasculature [198]. These proteins form the essence of what is commonly referred to as the Blood Brain Barrier (BBB). The proteins of the BBB involve structural proteins that line the intercellular walls between endothelial cells and form physical barriers (tight junctions) to prevent paracellular diffusion of chemicals. The BBB also includes a set of proteins that enable transport of molecules into the endothelial cell from the lumen of the capillary for trafficking intracellularly and exit on the brain side. Conversely, the BBB includes a complementary set of proteins that enable transport of molecules to enter the endothelial cell from the brain for trafficking intracellularly to exit to the capillary lumen. Both forms are described as transcellular transport. Finally, the BBB also contains a specific set of proteins (ABC transporters) that essentially capture passively diffusing molecules as they enter the endothelial cell and efflux to the lumen of the capillary. These proteins are known as BBB efflux proteins. It has been appreciated for some time that a number of opioids are substrates for one specific member of this class of transporter, P-glycoprotein (P-gp) [199]. Evidence in support of this includes enhanced brain uptake of morphine, methadone, and fentanyl in P-gp knock-out mice following intra-arterial perfusion [200], or microdialysis [201]. It is known that P-gp both contributes to preventing entry of opioids circulating in the blood to the brain as well as efflux opioids circulating in the CNS extracellular space to the blood [202]. Additionally, another member of the ABC BBB transporter family, the multidrug resistance protein (MRP), has recently been shown to similarly reduce the BBB transport of morphine from the systemic circulation to the CNS and to efflux morphine from the brain to the blood [203]. Specifically, it has been observed that antisense treatment to downregulate the expression of either P-gp [202] or MRP [203] or use of their respective knock-out mice resulted in enhanced analgesia induced by systemically administered morphine. This enhancement occurs presumably by enabling increased CNS levels of morphine. Interestingly, it has also be demonstrated that when opioids are delivered centrally, a component of the drug is efflux by BBB transport proteins to the periphery where activation of peripheral opioid receptors contributes to the overall analgesic effect synergistically with central opioid receptors [202]. Specifically, when p-glycoprotein [202] or MRP transporters [203] are reduced either by antisense or knock-out technology, the analgesic dose–response curves of intracerebroventricularly (i.c.v.) delivered morphine are shifted rightward, indicating diminished potency of the overall effect of i.c.v. morphine, presumably due to reduced efflux of morphine from the brain to the systemic circulation and, consequently, reduced activation of peripheral opioid receptors. These data are important observations in support the concept that P-gp and MRP also contribute to the efflux of opioids from the brain to the systemic circulation.
Analgesia of morphine [202, 204], fentanyl [204], and methadone [204] has been reduced in either P-gp KO mice or in the presence of P-gp inhibitors. It is, however, unclear what the therapeutic impact of P-gp influenced alterations in opioid concentrations may be. There is increasing evidence to suggest that P-gp levels are altered following a variety of environmental or physiological processes. Chronic administration of opioid has been reported to upregulate P-gp levels in a variety of tissues [205]; this would suggest that CNS concentrations under such conditions would be lowered and this may contribute to development of apparent opioid tolerance [206]. It would stand to reason that alterations in expression and/or function P-glycoprotein could arise under conditions of chronic pain that could influence the analgesic responsiveness of P-gp substrates, such as opioids. In fact, a study of peripheral inflammatory hyperalgesia using the standard intraplantar carrageenan model has demonstrated 40 % elevation in P-gp expression with a corresponding reduction in the antinociceptive efficacy of morphine [207]. Further, more detailed analysis has revealed that carrageenan-induced hypersensitivity results in protein trafficking alterations in P-gp at the level of the bilipid membrane [208]. Therefore, these studies provide the proof-of-concept that the condition of chronic pain can influence protein expression and function of P-gp. Peripheral inflammation is one model of chronic pain. More work is needed to understand the status of P-gp under a wide variety of pain conditions of diverse origin. There is clinical evidence to suggest that genetic variability in the ABC1 gene contributes to variability in patient responsiveness to opioids. Specifically it has been shown that methadone [209] or morphine [210] dosing for addiction and pain treatment respectively was associated with genetic variants of ABCB1, the gene that encodes P-gp. It seems that the interaction of the opioids with the P-gp and other transport proteins is highly complex involving both substrate interaction with P-gp and/or modulation of the expression of the P-gp system and/or patient genotype for the P-gp gene [205]. It would be greatly beneficial, therefore, to consider the status and impact of P-gp under the varied conditions of chronic pain and opioid self-administration models that are currently used separately and increasingly combined to further our understanding as to how subjects with established chronic pain respond to chronic opioid treatment.
Conclusion
When the condition of chronic pain is introduced into the complexity of the system, there are a number of points in the pharmacokinetic profile of each compound that may influence the reinforcing effects of a particular opioid. These aspects have been featured in this chapter. Although opioid receptor agonists are thought to converge on a common target, their pathways to the target, activities at the target, and retreat and distributions away from the target and out of the organism can be distinct. In experimental design and analysis of data, these considerations will be valuable to investigators intending to initiate studies of opioid self-administration in models of established chronic pain.
References
1.
IOM. Relieving pain in America: a blueprint for transforming prevention, care, education, and research. Washington, DC: The National Academies Press; 2011.
2.
Yekkirala AS, Banks ML, Lunzer MM, Negus SS, Rice KC, Portoghese PS. Clinically employed opioid analgesics produce antinociception via mu-delta opioid receptor heteromers in Rhesus monkeys. ACS Chem Neurosci. 2012;3:720–7.PubMedCentralPubMed
3.
Koob GF, Le Moal M. Neurobiological mechanisms for opponent motivational processes in addiction. Philos Trans R Soc Lond B Biol Sci. 2008;363:3113–23.PubMedCentralPubMed
4.
Calixto JB, Beirith A, Ferreira J, Santos AR, Filho VC, Yunes RA. Naturally occurring antinociceptive substances from plants. Phytother Res. 2000;14:401–18.PubMed
5.
Schug SA, Saunders D, Kurowski I, Paech MJ. Neuraxial drug administration: a review of treatment options for anaesthesia and analgesia. CNS Drugs. 2006;20:917–33.PubMed
6.
Hardman JG, Limbird LE. Goodman and Gilman’s the pharmacological basis of therapeutics. 9th ed. New York: McGraw Hill; 1996.
7.
Soars MG, Petullo DM, Eckstein JA, Kasper SC, Wrighton SA. An assessment of UDP-glucuronosyltransferase induction using primary human hepatocytes. Drug Metab Dispos. 2004;32:140–8.PubMed
8.
Green MD, King CD, Mojarrabi B, Mackenzie PI, Tephly TR. Glucuronidation of amines and other xenobiotics catalyzed by expressed human UDP-glucuronosyltransferase 1A3. Drug Metab Dispos. 1998;26:507–12.PubMed
9.
Stone AN, Macekenzie PI, Galetin A, Houston JB, Miners JO. Isoform selectivity and kinetics of morphine 3- and 6-glucuronidation by human UDP glucuronosyltransferases: evidence for atypical glucuronidation kinetics by UGT2B7. Drug Metab Dispos. 2003;31:1086–9.PubMed
10.
King CD, Rios GR, Assouline JA, Tephly TR. Expression of the UDP glucuronosyltransferases (UGTs) 2B7 and 1A6 in the human brain and identification of 5-hydroxytryptamine as a substrate. Arch Biochem Biophys. 1999;365:156–62.PubMed
11.
Yamada H, Ishii K, Ishii Y, Ieiri I, Nishio S, Morioka T, Oguri K. Formation of highly analgesic morphine-6-glucuronide following physiologic concentration of morphine in human brain. J Toxicol Sci. 2003;28:395–401.PubMed
12.
Congiu M, Mashford ML, Slavin JL, Desmond PV. UDP glucuronosyltransferase mRNA levels in human liver disease. Drug Metab Dispos. 2002;30:129–34.PubMed
13.
Deneau G, Yanagita T, Seevers MH. Self-administration of psychoactive substances by the monkey. Psychopharmacologia. 1969;16:30–48.PubMed
14.
Hill RC, Romer D. Comparison of the self-administration liabilities of morphine and pentazocine in the rhesus monkey. Br J Pharmacol. 1976;58:270P.PubMedCentralPubMed
15.
Mello NK, Mendelson JH. Effects of the neuropeptide DG-AVP on morphine and food self-administration by dependent rhesus monkey. Pharmacol Biochem Behav. 1979;10:415–9.PubMed
16.
Hoffmeister F. Progressive-ratio performance in the rhesus monkey maintained by opiate infusions. Psychopharmacology (Berl). 1979;62:181–6.
17.
Dewey WL. Background on animal testing in the drug delivery systems program. NIDA Res Monogr. 1981;28:69–76.PubMed
18.
van Ree JM, Slangen JL, de Wied D. Intravenous self-administration of drugs in rats. J Pharmacol Exp Ther. 1978;204:547–57.PubMed
19.
Collins RJ, Weeks JR, Cooper MM, Good PI, Russell RR. Prediction of abuse liability of drugs using IV self-administration by rats. Psychopharmacology (Berl). 1984;82:6–13.
20.
Shaham Y, Alvares K, Nespor SM, Grunberg NE. Effect of stress on oral morphine and fentanyl self-administration in rats. Pharmacol Biochem Behav. 1992;41:615–9.PubMed
21.
Shaham Y, Klein LC, Alvares K, Grunberg NE. Effect of stress on oral fentanyl consumption in rats in an operant self-administration paradigm. Pharmacol Biochem Behav. 1993;46:315–22.PubMed
22.
Garcia-Lecumberri C, Torres I, Martin S, Crespo JA, Miguens M, Nicanor C, Higuera-Matas H, Ambrosio E. Strain differences in the dose − response relationship for morphine self-administration and impulsive choice between Lewis and Fischer 344 rats. J Psychopharmacol. 2011;25:783–91.PubMed
23.
Semenova S, Danysz W, Bespalov A. Low-affinity NMDA receptor channel blockers inhibit acquisition of intravenous morphine self-administration in naive mice. Eur J Pharmacol. 1999;378:1–8.PubMed
24.
Carney JM, Landrum RW, Cheng MS, Seale TW. Establishment of chronic intravenous drug self-administration in the C57BL/6J mouse. Neuroreport. 1991;2:477–80.PubMed
25.
Brown RM, Stagnitti MR, Duncan JR, Lawrence AJ. The mGlu5 receptor antagonist MTEP attenuates opiate self-administration and cue-induced opiate-seeking behaviour in mice. Drug Alcohol Depend. 2012;123:264–8.PubMed
26.
Ambrosio E, Goldberg SR, Elmer GI. Behavior genetic investigation of the relationship between spontaneous locomotor activity and the acquisition of morphine self-administration behavior. Behav Pharmacol. 1995;6:229–37.PubMed
27.
Martın S, Manzanares J, Corchero J, Garcia-Lecumberri C, Crespo JA, Fuentes JA, Ambrosio E. Differential basal proenkephalin gene expression in dorsal striatum and nucleus accumbens, and vulnerability to morphine self-administration in Fischer 344 and Lewis rats. Brain Res. 1999;821:350–5.PubMed
28.
Belknap JK, Crabbe JC, Riggan J, O’Toole LA. Voluntary consumption of morphine in 15 inbred mouse strains. Psychopharmacology (Berl). 1993;1993:352–8.
29.
Milligan ED, Langer SJ, Sloane EM, He L, Wieseler-Frank J, O’Connor K, Martin D, Forsayeth JR, Maier SF, Johnson K, Chavez RA, Leinwand LA, Watkins LR. Controlling pathological pain by adenovirally driven spinal production of the anti-inflammatory cytokine, interleukin-10. Eur J Neurosci. 2005;21:2136–48.PubMed
30.
Semenova S, Kuzmin A, Zvartau E. Strain differences in the analgesic and reinforcing action of morphine in mice. Pharmacol Biochem Behav. 1995;50:17–21.PubMed
31.
Yoon YW, Lee DH, Lee BH, Chung K, Chung JM. Different strains and substrains of rats show different levels of neuropathic pain behaviors. Exp Brain Res. 1999;129:167–71.PubMed
32.
Mogil JS, Wilson SG, Bon K, Lee SE, Chung K, Raber P, Pieper JO, Hain HS, Belknap JK, Hubert L, Elmer GI, Chung JM, Devor M. Heritability of nociception I: responses of 11 inbred mouse strains on 12 measures of nociception. Pain. 1999;80:67–82.PubMed
33.
Betourne A, Familiades J, Lacassagne L, Halley H, Cazales M, Ducommun B, Lassalle JM, Zajac JM, Frances B. Decreased motivational properties of morphine in mouse models of cancerous- or inflammatory-chronic pain: implication of supraspinal neuropeptide FF(2) receptors. Neuroscience. 2008;157:12–21.PubMed
34.
Suzuki T, Kishimoto Y, Misawa M. Formalin- and carrageenan-induced inflammation attenuates place preferences produced by morphine, methamphetamine and cocaine. Life Sci. 1996;59:1667–74.PubMed
35.
Narita M, Kishimoto Y, Ise Y, Yajima Y, Isawa K, Suzuki T. Direct evidence for the involvement of the mesolimbic kappa-opioid system in the morphine-induced rewarding effect under an inflammatory pain-like state. Neuropsychopharmacology. 2005;30:111–8.PubMed
36.
Ozaki S, Narita M, Narita M, Ozaki M, Khotib J, Suzuki T. Role of extracellular signal-regulated kinase in the ventral tegmental area in the suppression of the morphine-induced rewarding effect in mice with sciatic nerve ligation. J Neurochem. 2004;88:1389–97.PubMed
37.
Niikura K, Narita M, Narita M, Nakamura A, Okutsu D, Ozeki A, Kurahashi K, Kobayashi Y, Suzuki M, Suzuki T. Direct evidence for the involvement of endogenous beta-endorphin in the suppression of the morphine-induced rewarding effect under a neuropathic pain-like state. Neurosci Lett. 2008;435:257–62.PubMed
38.
Ozaki S, Narita M, Narita M, Iino M, Sugita J, Matsumura Y, Suzuki T. Suppression of the morphine-induced rewarding effect in the rat with neuropathic pain: implication of the reduction in mu-opioid receptor functions in the ventral tegmental area. J Neurochem. 2002;82:1192–8.PubMed
39.
Ozaki S, Narita M, Narita M, Iino M, Miyoshi K, Suzuki T. Suppression of the morphine-induced rewarding effect and G-protein activation in the lower midbrain following nerve injury in the mouse: involvement of G-protein-coupled receptor kinase 2. Neurosci Lett. 2003;116:89–97.
40.
Petraschka M, Li S, Gilbert T, Westenbroek R, Bruchas M, Schreiber S, Lowe J, Low M, Pintar J, Chavkin C. The absence of endogenous beta-endorphin selectively blocks phosphorylation and desensitization of mu opioid receptors following partial sciatic nerve ligation. Neuroscience. 2007;146:1795–807.PubMedCentralPubMed
41.
Oe K, Narita M, Imai S, Shiasaki M, Kubota C, Kasukawa A. Inhibition of the morphine-induced rewarding effect by direct activation of spinal protein kinase C in mice. Psychopharmacology (Berl). 2004;177:55–60.
42.
Shippenburg TS, Stein C, Huber A, Millan MJ, Herz A. Motivational effects of opioids in an animal model of prolonged inflammatory pain: alteration in the effects of kappa but not of mu receptor agonists. Pain. 1988;35:179–86.
43.
Sufka KJ. Conditioned place preference: a novel approach for analgesic drug assessment against chronic pain. Pain. 1994;58:355–66.PubMed
44.
Cahill CM, Xue L, Grenier P, Magnussen C, Lecour S, Olmstead MC. Changes in morphine reward in a model of neuropathic pain. Behav Pharmacol. 2013;24:207–13.PubMed
45.
Martin TJ, Kim SA, Buechler NL, Porreca F, Eisenach JC. Opioid self-administration in the nerve-injured rat: relevance of antiallodynic effects to drug consumption and effects of intrathecal analgesics. Anesthesiology. 2007;106:312–22.PubMed
46.
McWilliams K, Fallon M. Fast-acting fentanyl preparations and pain management. QJM. 2013;106:887–90.PubMed
47.
Paech MJ, Bloor M, Schug SA. New formulations of fentanyl for acute pain management. Drugs Today (Barc). 2012;48:119–32.
48.
Davis MP. Fentanyl for breakthrough pain: a systematic review. Expert Rev Neurother. 2011;11:1197–216.PubMed
49.
Sellers EM, Schuller R, Romach MK, Horbay GL. Relative abuse potential of opioid formulations in Canada: a structured field study. J Opioid Manag. 2006;2:219–27.PubMed
50.
Edinboro LE, Poklis A, Trautman D, Lowry S, Backer R, Harvey CM. Fatal fentanyl intoxication following excessive transdermal application. J Forensic Sci. 1997;42:741–3.PubMed
51.
Liappas IA, Dimopoulos NP, Mellos E, Gitsa OE, Liappas AI, Rabavilas AD. Oral transmucosal abuse of transdermal fentanyl. J Psychopharmacol. 2004;18:277–80.PubMed
52.
Woodall KL, Martin TL, McLellan BA. Oral abuse of fentanyl patches (Duragesic): seven case reports. J Forensic Sci. 2008;53:222–5.PubMed
53.
Barrueto Jr F, Howland MA, Hoffman RS, Nelson LS. The fentanyl tea bag. Vet Hum Toxicol. 2004;46:30–1.PubMed
54.
Coon TP, Miller M, Kaylor D, Jones-Spangle K. Rectal insertion of fentanyl patches: a new route of toxicity. Ann Emerg Med. 2005;46:473.PubMed
55.
Feierman DE, Lasker JM. Metabolism of fentanyl, a synthetic opioid analgesic, by human liver microsomes. Role of CYP3A4. Drug Metab Dispos. 1996;24:932–9.PubMed
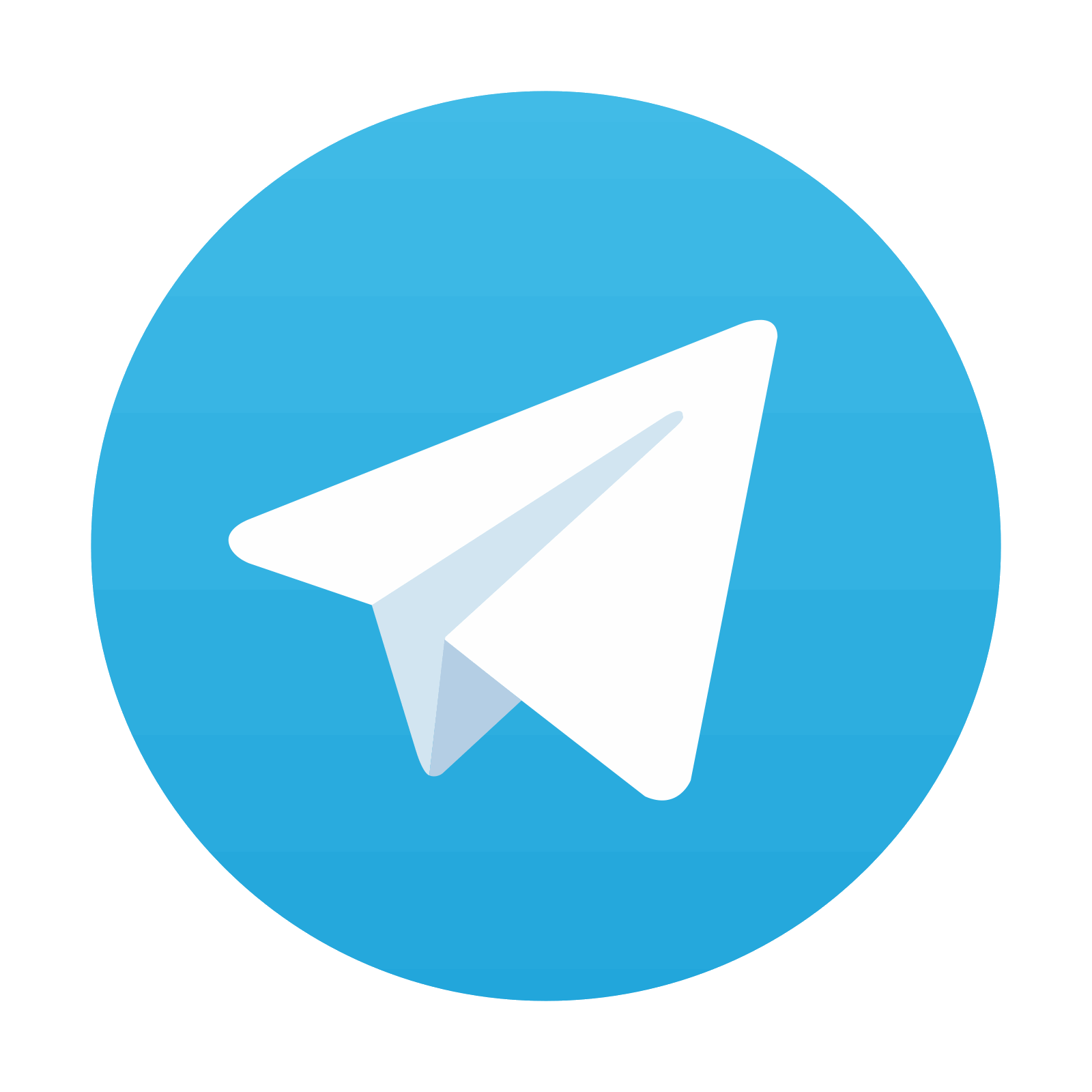
Stay updated, free articles. Join our Telegram channel
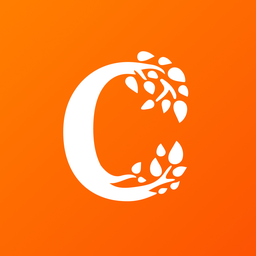
Full access? Get Clinical Tree
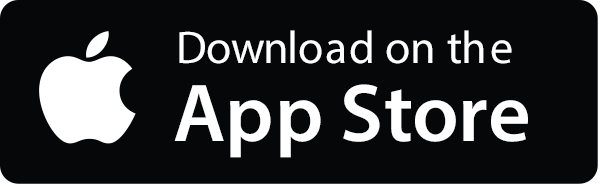
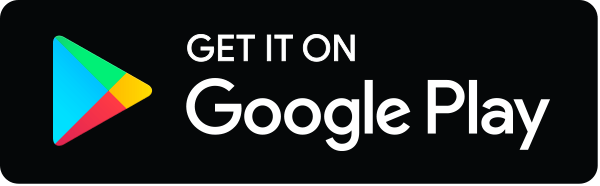