Fig. 1
Starling sleep. A 40-s trace of EEG and EOG recordings from a sleeping starling is shown. The first ~10 s of the EEG signal shows low-frequency, high-amplitude waves characteristic of slow-wave sleep with minimal eye movement in the EOG signal. The EEG signal then rapidly transitions to high-frequency, low-amplitude waves characteristic of REM sleep with associated rapid-eye movements appearing in the EOG signal seconds later (T. Brawn, unpublished data)
REM sleep in birds is characterized by a desynchronized low-amplitude, high-frequency EEG pattern similar to the waking state. While rapid-eye movements during REM sleep are common, the muscle atonia that is a defining feature of REM sleep in most mammals is less consistent in birds. Additionally, the duration of REM sleep episodes is shorter in birds than in mammals, lasting from a few to tens of seconds. The brief duration of REM sleep episodes has likely led to an underreporting of REM sleep in earlier investigations of avian sleep (see Lesku et al. 2009 for a discussion of scoring sleep states in birds). For instance, REM sleep in the zebra finch was originally reported as averaging 0.2 h per 24-h period (Schmidt et al. 1990). In contrast, a more recent study that included sophisticated automated techniques confirmed by extensive manual scoring reported that zebra finches spent 23 % of an 8-hour dark period in REM sleep (Low et al. 2008). Like mammals, the amount of REM sleep is relatively low early in the night and increases as sleep continues (Low et al. 2008; Martinez-Gonzalez et al. 2008).
The few recent studies of avian sleep have shown a systematic variation of SWS and REM sleep across the night. Indeed, a study of sleep in adult male zebra finches found an ultradian pattern characterized by a decrease in SWS (from 50 to 25 %) across the night in conjunction with an increase in the percentage of REM sleep (from 8 to 30 %) as well as an increase in the average duration of REM sleep bouts (from 7 to 15 s) (Low et al. 2008). Preliminary results (T. Brawn, unpublished) indicate similar dynamics including a high density of REM sleep in another passerine species, the European starling , which contrasts with earlier studies of starling sleep (Szymcazk 1985, 1986). Whether there is a difference between songbirds and non-passerine species remains unresolved. A recent molecular phylogeny places psittaciformes (parrots) as the sister group to passerines (Hackett et al. 2008). Though the only studies of parakeet sleep reported very low levels of REM (Ayala-Guerrero et al. 1988; Ayala-Guerrero 1989), the recordings were made under conditions of constant (24 h) light, which may not be representative of sleep in birds experiencing a natural light/dark cycle.
The patterns of avian sleep in recent studies are largely consistent with how sleep is described in mammals. However, it is necessary to recall that there is no single pattern of sleep for mammals; rather there are vast differences in the duration and structure of sleep across the range of mammalian species. This is obvious even when only considering the most common subjects of mammalian sleep studies: rats, cats, and humans. Such considerations indicate a compelling need for additional comparative work in birds, especially when considering that many studies of avian sleep were conducted decades ago and will likely need to be revised to reflect advances in recording technologies, computational methods for sleep-stage analysis, and a greater understanding of the structure of sleep in birds.
4 An Introduction to the Birdsong System
There are ~10,000 species of birds. Whereas all birds can produce innate calls, only songbirds (~4,000 species), parrots (~350 species), and hummingbirds (~350 species) can learn to produce vocalizations. Studies in the songbird species zebra finch (Taeniopygia guttata) have provided the most extensive evidence in birds linking sleep to learning and memory consolidation. All true songbirds (i.e., oscine passerines) express specialized forebrain nuclei necessary for song learning and production. The “motor” pathway, which is required for singing, consists of direct projections from the sensorimotor cortical nucleus HVC to the motor output nucleus RA. The “anterior forebrain pathway” (AFP), which is required for song learning, also begins in HVC and ends with projections to RA. This pathway, however, additionally includes the basal ganglia components of the song system in Area X (Farries and Perkel 2002; Carrillo and Doupe 2004; Farries et al. 2005) and represents a cortico-basal ganglia-thalamocortical pathway. The AFP, which gives rise to variability in vocalizations (Sohrabji et al. 1990; Scharff and Nottebohm 1991; Kao et al. 2005; Ölveczky et al. 2005), is the primary source of RA excitation during early development. This likely explains the highly variable vocalizations that are produced in young juveniles (Aronov et al. 2008). The influence of the AFP on song production, however, changes during development, with the AFP having a minimal direct influence on adult singing. The AFP, nonetheless, is involved in regulating auditory feedback (Brainard and Doupe 2000) and may contribute to the sleep-related regulation of singing (Andalman and Fee 2009). After receiving input from the motor and AFP pathways, RA projects to brainstem structures involved in syringeal and respiratory control. These brainstem structures, along with higher-order auditory structures, feedback onto HVC. Neurons in RA have been the focus of several studies relating song system physiology with singing behavior and are known to express sleep-dependent features, as described below.
5 Sleep and Developmental Song Learning in Zebra Finches
The song learning process in zebra finches begins during the altricial phase of development. During this phase of song learning, a juvenile zebra finch forms a sensory memory of the “tutor” song produced by its father or a different adult male conspecific. This sensory memory has traditionally been viewed as a “template” or comparator that guides vocal learning (Adret 2004). In spite of extensive research effort, unambiguous identification of this comparator remains unresolved. Physiological processes related to the template include immediate early gene (IEG) expression and related signaling pathways in the auditory system early in development (Terpstra et al. 2005; London and Clayton 2008), single neuron selectivity for tutor song in HVC early in development (Adret et al. 2012), single neuron selectivity for tutor song in the AFP at intermediate stages of development (Solis and Doupe 1999), reversible lesion studies of the AFP (Aamodt et al. 1996), and others. Gobes et al. (2010) demonstrated IEG expression related to tutor song acquisition but only in higher-order auditory areas. No expression was detected in song system nuclei. When single neuron recordings were made in the HVC of presinging birds, however, a small population of neurons was detected that was selective to the tutor song (Adret et al. 2012). The selectivity of those neurons for song was highly significant compared to other adult conspecific songs including the familiar songs of birds from neighboring cages. Such a small population would be difficult to detect in bulk IEG expression studies.
Collectively, these results indicate a broad distribution of structures expressing effects of tutor song exposure. This argues that even if there is a single structure that acts as the primary site of the template, this memory is rapidly distributed throughout the song system . Such memory transference is a hallmark of sleep-dependent memory consolidation, and it is a viable conjecture that memorization of the tutor song is a sleep-dependent phenomenon. Whereas a role for sleep in the sensory memory formation of the tutor song has not been directly tested, nonetheless, isolated juveniles have been observed to rapidly fall asleep upon their first exposure to a singing adult (T. Lints, unpublished data from the Tchernichovski lab; P. Adret, unpublished data). Though speculative, this supports the suggestion that sleep could be important to this process and would be consistent with sleep consolidation studies of sensory memory in humans.
The first song-related vocalizations begin during the sensorimotor phase of song learning. Initially, juvenile birds produce subsong, which consists of relatively long and variable sequences of unstructured sounds, a process similar to babbling in human infants. Despite the relative lack of structure in subsong, these vocalizations have greater spectrotemporal complexity and variability than innate calls, which are distinct from song. Subsong transitions into plastic song, in which vocalizations become more structured such that sequences of individual syllables become recognizable but lack the stereotypy of adult song. The plastic song continues to be modified as it approaches an increasingly accurate imitation of the tutor song. The bird finally produces a crystallized song, which is characterized by temporal and spectral stereotypy, at the time of sexual maturity. The process of developmental song learning has been extensively studied, and numerous detailed reviews are available (e.g., Marler 1997; Margoliash 2002; Lipkind and Tchernichovski 2011).
Although song learning has long been studied, compelling evidence that sleep plays a critical role in the sensorimotor phase of song learning has only recently been described, and to date, this has only been established in zebra finches . To bring the process of developmental song learning under experimental control, a juvenile bird is raised by its non-singing mother in a sound isolation chamber to enable high-quality vocal recordings. When the juvenile becomes independent, it is given access to a tutor song by pecking a key or pulling a string (Tchernichovski et al. 2001, 2004). Though this differs from the natural developmental process in which zebra finches live in groups and learn from adult male tutors, this paradigm controls for the variation in singing behavior by the tutor and the social interactions between the juvenile and tutor that would otherwise occur. A juvenile bird in this paradigm is first exposed to a tutor song at ~40 days post-hatch.
A circadian variation in singing rapidly emerges such that vocalizations early in the day have less structure than vocalizations later in the day, a pattern that continues but attenuates throughout the song learning process. Accordingly, songs that are produced in the afternoon have more spectrotemporal complexity and are more accurate copies of the tutor song than vocalizations from the previous or subsequent mornings. Moreover, preventing juveniles from singing in the morning results in songs with less structure when they are allowed to sing in the afternoon. Conversely, a bout of sleep during the day leads to a second round of lower structure songs. Thus, sleep is affecting behavior in a seemingly inverse fashion, whereby the songs sung early in the day are less complex than songs produced later in the day. This circadian pattern is directly related to sleep and is instrumental in the song learning process. Critically, juveniles that ultimately produce the most accurate copies of the tutor song are those that had that greatest magnitude of circadian variation during the sensorimotor phase of song learning (Derégnaucourt et al. 2005). This result seems counter-intuitive to the typical effects of sleep on learning and memory. However, juvenile vocalizations are highly variable, especially early in development. Because the song learning process involves extensive daily practice over ~60 days, the reduction in song complexity across sleep may be a mechanism to prevent juvenile birds from prematurely crystalizing song features that do not accurately represent the tutor song. The pattern itself may arise as an interaction between sensory memories and motor memories, both of which may be changing as birds learn to sing (see below). This emphasizes the sensorimotor nature of birdsong learning, which is distinct from the perceptual, procedural, or episodic memories more commonly studied in humans.
Examining the firing properties of RA (primary motor cortex analog) during sleep has further confirmed a role for sleep in song learning. Prior to tutor song exposure, neurons in RA expressed low spontaneous rates with little bursting, which could be represented by a unimodal interspike interval (ISI) histogram. However, the ongoing discharge profile of RA was significantly altered on the first night of sleep after tutor song exposure. There was an increase in short-interval ISIs, many of which were organized into protobursts, and the ISI histogram became bimodal. It seems plausible that the sleep bursting in RA is part of the causal mechanism driving the circadian variation in singing because the circadian singing pattern only materialized on the morning after the sleep bursting emerged. The birds in these experiments were exposed to one of three different tutor songs, and the average ISI histogram for each bird was similar for birds trained on the same tutor song and different from birds trained on the other tutor songs. The shape of the ISI histogram, however, was dynamic such that the firing properties of RA during sleep exhibited corresponding changes in the ISI histograms when birds were switched from one tutor song to another. The dynamic nature of the ISI distributions that characterize the different tutor songs suggests that the information represented in the sleep bursting was directly related to the tutor song rather than to the subsong vocalizations of the juvenile birds (Shank and Margoliash 2009). RA neurons begin to express auditory responses during sleep only once a juvenile bird transitions from subsong to plastic song (M. Lusignan, unpublished data), which also coincides with HVC taking over from the AFP as the predominant driver of RA activity. At this point in development, RA responds selectively to the playback of the bird’s own song during sleep.
The effect of sleep (as assessed by depth of circadian variation) is strongest when RA is driven by the AFP and when singing is most variable. The nighttime activity of the RA motor neurons at this early time in development expresses the sensory memory. A theory that consolidates all these observations is not yet within reach. But we note that in contrast to other systems, this one expresses the interactions of sensory and motor memories, both changing over the time course of development. We speculate that these two memories both induce changes in singing behavior but at different phases (sleep and singing), and the interaction between the two processes is less coordinated early in development. We further speculate that the sensorimotor mappings of HVC-RA pass a critical threshold that enables populations of HVC-RA neurons to provide structured input into RA during sleep, which transmits auditory information from HVC to RA and drives sleep bursting starting at the onset of plastic singing.
Finally, song learning in zebra finches is not the only learning phenomenon in juvenile birds for which sleep is essential. The consolidation of visual imprinting in the (non-songbird) domestic chick (Gallus gallus domesticus) also relies on sleep. Young visually naïve chicks can learn the visual characteristics of a conspicuous object (i.e., the imprinting stimulus, or IS) upon exposure. Once imprinted, chicks will approach and direct social behaviors toward the imprinted object but not toward novel objects. After exposure, IS-selective neurons begin to emerge in the intermediate and medial mesopallium. In one study, the maximal proportion of IS-selective neurons was observed at 25 h after IS exposure. The largest increase in IS-selective neurons occurred between 8 and 25 h after exposure, which indicated a possible role of sleep in the consolidation of the IS memory (Horn et al. 2001). Later work confirmed the necessity of sleep. Indeed, chicks that were allowed undisturbed sleep after exposure had significantly more IS-selective neurons at 19.5 h post-exposure than chicks whose undisturbed sleep was delayed by 7.5 h. Though sleep states were not scored, an increase in EEG power in low frequencies (0–6 Hz) was observed during the sleep period. Furthermore, only chicks that were allowed undisturbed sleep immediately after the IS exposure expressed a behavioral preference for the IS when tested 20 h later (Jackson et al. 2008). Overall, studies of juvenile zebra finches and domestic chicks have demonstrated an essential role of sleep in the consolidation of two distinct critical-period learning phenomena, which shares similarities with the effects of sleep on language-learning tasks in human infants (Gomez et al. 2006; Hupbach et al. 2009).
6 Sleep and Adult Song Maintenance in Zebra Finches
Skilled behaviors require continued practice to be maintained at a high level of performance, a process that perhaps involves sleep. Zebra finches produce remarkably stereotyped songs throughout their adult lives. One explanation for this consistency is that zebra finches perform their song extensively each day. Another contributing factor may be subtle modifications to the firing properties of RA neurons during sleep that affect daytime singing behavior.
One proximate mechanism for regulating daytime behavior by nighttime processes is modification of neuronal replay. Neuronal replay refers to offline activity of single neurons or neural populations that produce approximate copies of the patterns of neuronal activity observed during waking behavior. Though replay can occur during wakefulness, it has most commonly been detected during sleep. To demonstrate sleep replay, it is necessary to compare neural activity during a specific behavior with the neural activity of the same neuron or population during sleep. Perhaps the most compelling example of veridical sleep replay at a single neuron level is that of RA neurons in adult zebra finches (Dave and Margoliash 2000) (Fig. 2). These neurons, which emit precisely timed bursts every time a motif is sung, occasionally burst while zebra finches sleep. Bursts occurring at different parts of the song have differences in the number or timing of spikes within the bursts. Because the variation of spikes within a burst associated with one part of the song is much lower than the differences in bursts related to other parts of the song, RA neurons have unique bursts, which has facilitated the detection and analysis of neural replay during sleep. In a study of sleep replay, a burst was defined as a sequence of interspike intervals that fell outside the non-bursting distribution. Approximately 7 % of RA spikes during sleep were part of a burst, and 15 % of sleep bursts matched a burst that the neuron emitted while the bird was singing (Dave and Margoliash 2000). These estimates, however, represent a lower bound on sleep bursting because for statistical reasons only long bursts (8 or more spikes) were analyzed. Moreover, a sleep burst was only considered to match a singing burst if the precise timing of individual spikes within the bursts matched, yet the relation between singing and sleeping bursts was often compelling even if a subset of spikes within a burst differed. Furthermore, bursting tends to synchronously recruit many neurons within the song system , which suggests that the amount of RA sleep bursting that represents replay would be higher with simultaneous single-cell recordings of a population of RA neurons.


Fig. 2
Neuronal replay in the zebra finch song system . A spectrogram (frequency vs. time) of zebra finch song is shown in the top panel, with two 900-ms raw traces of activity of a single RA neuron below. The top trace of neuronal activity was recorded at the same time the bird sang the motif displayed above it. Note the bursting activity, which is characteristic of RA activity during singing. The bottom neuronal trace is of spontaneous discharge activity recorded while the bird slept at night. Note that after three single spikes (typical of background or resting activity during sleep), the neuron began to burst, with the timing and structure of the bursts closely approximating the activity of the neuron during singing. A statistical analysis of individual spike bursts (not considering trains of spike bursts such as are displayed in the figure) demonstrated that such matches occurred at a statistically significant level in 13/14 neurons tested (Dave and Margoliash 2000). Scale bar, 100 ms
The replay of a specific waking behavior indicates that precise information is represented in neural activity during sleep. If sleep replay is a mechanism of memory consolidation, then patterns of neural activity should change across sleep in a manner that is adaptive to learning and memory. Taking advantage of the reliability of RA activity during singing, a recent study reported systematic changes in the bursting properties of individual neurons that were recorded during singing and that were maintained across periods of sleep (Rauske et al. 2010). The structure of 33 of 115 bursts (28.7 %) changed across sleep. By comparison, only 18 of 551 bursts (3.3 %) exhibited similar changes across daytime singing. The primary change was a loss in the number of spikes per burst after sleep (Fig. 3), yet the interspike intervals for the altered bursts increased such that the overall burst duration remained approximately constant. Half of the recorded neurons expressed at least one burst change when comparing pre- and post-sleep singing.


Fig. 3
RA neurons lose spikes across sleep. An example of changes across sleep in the pattern of activity of one burst from an RA neuron is shown. Each tick mark represents the timing of one spike, relative to a zero time established to align the data across multiple renditions of the motif that the bird sang. The burst comprises multiple spikes emitted over a period of approximately 15 ms. The neuron was recorded for 20 renditions of the motif prior to when the bird slept for approximately 140 min (dashed line). The neuron was then recorded for an additional 25 motifs after the bird awoke and began to sing again. Note that the third spike (at the arrow) in the pre-sleep structure of the burst is missing in the post-sleep structure of the burst, representing a change in burst structure and a loss in spikes following sleep
Though the changes to burst structure may be subtle, the accumulated changes over one night, let alone over the lifespan of the bird, would drastically impair a zebra finch’s song if there were not a compensatory response to add spikes back into the population. Given the stability of adult zebra finch songs, how do spikes get back into the system? This is the subject of speculation because to date spike gain has yet to be experimentally observed. On the one hand, zebra finches sing their unique motifs (relatively fixed sequences of syllables) upward of 1,000 times per day. This intensive practice likely potentiates the synapses in RA, which could lead to an increase in the number of spikes emitted. On the other hand, neurogenesis is known to take place within the song system , specifically involving neurons projecting form HVC to RA (Goldman and Nottebohm 1983). New neurons, which are initially incorporated into local HVC circuits (Paton and Nottebohm 1984), project to and synapse in RA in 1–2 weeks (Alvarez-Buylla et al. 1988). A steady addition of synapses from HVC could also counteract the nightly loss of spikes in RA neurons. This phenomenon of losing spikes across sleep and gaining spikes across wakefulness may represent an instantiation at the single neuron level of the synaptic homeostasis hypothesis (Tononi and Cirelli 2003, 2006). Alternatively, each night many neurons in RA may lose spikes (loss of drive from HVC), but only some would be driven below a threshold where they can accept new inputs from HVC (perhaps arising from afferents of recently born HVC neurons recently arrived in RA). Under this hypothesis, if the number of neurons losing spikes at night were much greater than the number gaining spikes at night, the latter would be difficult to detect in challenging physiological recordings.
The loss of spikes within RA bursts was first observed during sleep rather than when the bird began singing in the morning. The structure of the modified sleep bursts was more similar to the bursts that would occur during singing on the following day compared to the bursts that occurred during singing on the previous day (Z. Chi, unpublished results). Accordingly, the activity of RA during sleep includes a “preplay” component that is predictive of future neural activity rather than a recapitulation of past activity. This finding of preplay coincides with recent work in the rodent hippocampal system that has also uncovered instances of sleep preplay (Dragoi and Tonegawa 2011, 2013). This suggests the need for a reevaluation of the literature on offline reactivations to determine whether replay and preplay are distinct phenomena or whether the many reports of reactivation of previous waking behavior would be more accurately described as preplay of future waking activity.
The study of RA activity during sleep offers a significant opportunity that is largely untapped to explore the development and function of sleep replay in relation to a known and stable behavior (i.e., the bird’s stereotyped song). The presence of sleep replay (or preplay) in RA also indicates that the reactivation of waking neural activity during sleep extends beyond the hippocampal memory system and therefore may be a mechanism of memory consolidation that generalizes to other memory systems. Nonetheless, the studies of sleep replay in zebra finches share an important weakness with the studies of rodent sleep reactivation. Namely, the replay phenomenon in RA is not directly connected to behavioral evidence of learning and memory. In juveniles, the sleep bursting activity in RA is not necessarily a replay of waking activity. Indeed, the lack of song stereotypy and precise RA activity make detecting replay at this stage of development difficult, but it also offers an opportunity to track the emergence of replay phenomena in a developing system. Likewise, there is not a direct connection between sleep replay and song maintenance in adults. From the neural perspective, the replay of singing neural activity occurs during sleep, and the bursting properties of individual neurons change across sleep. From the behavioral perspective, adult zebra finch song remains remarkably stable from day to day. Nonetheless, a causal relationship between replay (or preplay) and the maintenance of adult song has yet to be fully established.
7 A New Animal Model of Sleep Consolidation: Auditory Classification Learning in European Starlings
A dichotomy between human and animal research of sleep consolidation has persisted. Human studies have provided convincing behavioral evidence that retention intervals with sleep benefit memory compared to similar periods of wakefulness. In conjunction with imaging and polysomnography, human studies have additionally been able to localize memory representations to specific brain regions, identify how those memories are reorganized across brain regions after sleep, and relate performance changes to specific electrophysiological features of sleep. Nonetheless, human studies are limited at determining the underlying neuronal, molecular, and genetic mechanisms that are responsible for sleep-dependent memory benefits. Animal studies, on the other hand, have provided data that could be the underlying basis of sleep consolidation. While sleep deprivation has been shown to impair memory consolidation in animals, there is still limited behavioral evidence showing that sleep, compared to natural wakefulness, benefits recently acquired memories in adult animals as is commonly observed in humans. To address this, we developed an auditory classification learning paradigm in European starlings (Sturnus vulgaris) that was modeled after the standard behavioral approach of human studies of sleep consolidation.
Starlings are a songbird species with complex vocalizations consisting of long sequences of temporally discrete motifs, with each motif lasting ~1 s and being composed of a sequence of shorter syllables (Adret-Hausberger and Jenkins 1988; Eens 1997). Whereas zebra finches only learn a single motif that becomes crystallized around 90 days post-hatch, starlings are open-ended learners that continue to add new motifs to their repertoire throughout their lives, including the incorporation of sounds produced by different species of songbirds, other animals, and environmental noises. A bout of starling song can be wondrously complex, but tends to follow an overall structure. Starlings begin with introductory notes followed by a sequence of some of the motifs within a starling’s repertoire, with each motif being produced one or more times. “Warbles” (a category of motifs) tend to appear earlier in a song bout, followed by a transition to the “rattle” category of motifs, and singing often terminates with loud high-frequency whistles. A single song bout may last between 30 and 120 s, and no two song bouts are likely to be the same.
Wild starlings maintain large repertoires of unique motifs, and starlings can learn to identify individuals by associating the production of certain motifs with specific individuals (Gentner and Hulse 2000; Gentner et al. 2000). Starlings are also very adaptable to laboratory caging and can be trained with operant techniques to classify auditory stimuli through differential reinforcement of responses to different stimuli. In the Go/No-Go paradigm, starlings are rewarded with food when they respond to the “Go” stimulus, whereas the cage lights are turned off when they respond to the “No-Go” stimulus. Over the course of training, starlings learn to respond to the Go stimulus and withhold response from the No-Go stimulus, demonstrating the ability to learn and maintain auditory classifications. The behavioral importance of song recognition for starlings along with the ease in which they can perform operant learning tasks in the laboratory makes starlings an attractive model system for studying auditory perceptual learning and associative memory.
In an early experiment, we investigated whether adult starlings express behavioral evidence of sleep consolidation in a manner similar to what has been observed in humans. The starlings first completed 4 conditions in which they learned to classify pairs of 5-s segments of novel starling song. The starlings were then tested immediately after the training session and again after a retention period that consisted of wakefulness or that included sleep. Classification performance in the “wake” condition, wherein starlings were trained in the morning and retested in the evening, decreased non-significantly from the post-training test to the post-retention test (Fig. 4a). In contrast, starlings in the “sleep” condition, which were trained in the evening and retested the next morning, exhibited a significant performance improvement across the night of sleep. Classification performance also improved significantly across 24-h retention intervals regardless of whether starlings were trained in the morning or evening. This pattern of results was then replicated in two conditions that entailed post-tests after both waking and sleeping retention. Classification performance in the “AM-PM-PM” condition decreased non-significantly across the day but improved significantly after sleep. Likewise, performance in the “PM-AM-PM” condition improved significantly after a night of sleep followed by a non-significant change across the next day. The results demonstrate that sleep produces a pattern of memory benefits in starlings that is similar to that observed in humans, providing clear behavioral evidence of sleep-dependent consolidation in an adult animal (Brawn et al. 2010a).


Fig. 4
Auditory classification performance improvement in starlings. Starlings were trained to classify two 5-s segments of novel starling song and retested after retention period that consisted of wakefulness (gray bars) or that included a night of sleep (black bars). Performance improvements scores were calculated as the difference between the post-retention and post-training test scores. Data are means ±SEM (*p < 0.05; **p < 0.01; ***p < 0.001). Data in part a are from Brawn et al. (2010a). Data in parts b and c are from Brawn et al. (2013)
One intriguing difference between the patterns of results in the starling and human studies is that task performance in humans often deteriorates significantly across the day (e.g., Fenn et al. 2003, 2013; Ellenbogen et al. 2006, 2009; Brawn et al. 2008, 2010b; Payne et al. 2008), whereas the performance decline across waking retention was not significant in the starlings. Though species or task-related differences could explain this inconsistency, this difference could also reflect the negative effects of interference in humans because daytime behavior in human studies is rarely controlled. The starlings , however, were isolated throughout the experiments and were only presented with a very familiar baseline stimulus set when they were not participating in training or testing sessions, thus greatly reducing potential sources of interference. To address this possibility, we expanded the auditory classification paradigm to explore the interaction between interference and consolidation across waking and sleeping retention by training starlings on two similar classification tasks (Brawn et al. 2013).
In this study, starlings each completed 7 experimental conditions that followed an A–B–A (interference) or A–A (control) design. Training and testing, as before, were accomplished using the Go/No-Go paradigm. In the wake-retention sessions, starlings were trained and tested on classification A in the morning and retested in the evening. Starlings were additionally trained on classification B, which consisted of a novel pair of starling song stimuli, immediately after completing task A (“Early Interference”) or 4 h later (“Late Interference”). In the sleep-retention sessions, starlings were trained and tested on task A and retested after a night of sleep. Similar to the wake-retention sessions, starlings were trained and tested on task B after completing task A (“Early Interference”), 4 h later (“Late Interference”), or on the next morning prior to the post-retention test (“Post-sleep Interference”). Two control conditions were also run (“Wake” and “Sleep” Control), which did not include task B interference. The “Wake Control” condition exhibited a non-significant performance decline across the day (Fig. 4b). Classification performance for task A, however, deteriorated significantly across waking retention in the “Early Interference” and “Late Interference” conditions. In contrast, classification performance improved significantly after sleep for the “Sleep Control” and the sleep interference conditions (“Early,” “Late,” and “Post-Sleep”). This pattern of results indicates that learning a second classification task retroactively interfered with the memory of classification A, resulting in significantly impaired performance after waking retention. Nonetheless, sleep consolidated the memory of classification A such that performance was enhanced and stabilized after sleep to the point of eliminating any measurable effect of interference (Brawn et al. 2013).
Knowing the fate of the memory for classification A, we conducted an additional experiment to determine whether the interfering material (i.e., classification task B) was also consolidated across sleep. In this experiment, starlings each completed 6 conditions that followed an A–B–B (interference) or B–B (control) design in which they were retested on task B rather than task A after waking or sleeping retention. In the Early Interference conditions, starlings were trained on task A in the morning and then immediately trained on task B. The “Early Wake” condition was then retested on task B in the evening, and the “Early Sleep” condition was retested on the following day. In the Late Interference conditions, starlings were trained on task A in the morning and then trained on task B 4 h later. The “Late Wake” condition was then retested on task B in the evening and the “Late Sleep” condition was retested on task B on the following day. Two wake control conditions (“Early” and “Late” Control) were only trained and tested on task B at the same times as the Early and Late Interference conditions, respectively. Classification performance in the “Early Control” and “Late Control” conditions, as before, exhibited a non-significant decline across their respective waking retention intervals (Fig. 4c). In contrast, task B performance significantly deteriorated across the same intervals in the “Early Interference” and “Late Interference” wake conditions, demonstrating that the learning of classification A prior to classification B proactively interfered with the memory of classification B at the end of the day. Despite this proactive interference, classification performance on task B was significantly improved after sleep in both the “Early Interference” and “Late Interference” sleep conditions (Brawn et al. 2013).
Together, these two interference studies reveal that the learning of each classification reliably interfered, retroactively and proactively, with the retention of the other classification across wakefulness. Sleep, however, consolidated the memories of both classifications even after performance was impaired by interference prior to sleep. Though interference increased the performance loss across waking retention, performance after sleep was no different in the interference and control conditions. Thus, as in the human studies of interference and sleep consolidation (e.g., Ellenbogen et al. 2006), the inclusion of the interfering material actually magnified the memory benefit of sleep. These observations demonstrate that sleep consolidation separately enhances memory of interfering experiences, facilitating opportunistic daytime learning. This system is ripe for further exploration: For example, “What types of memories interfere with each other?”; “Does the time course of performance loss or magnitude of interference depend on the complexity of the task?”; “Does the consolidated memory remain stable over many days?”; “Will the pattern of performance loss followed by post-sleep recovery be observed in other forms of associative memory formation?” and many others. Answers to these questions can help provide a rich behavioral framework for theory making as well as help to direct neurophysiological experiments designed to test those theories.
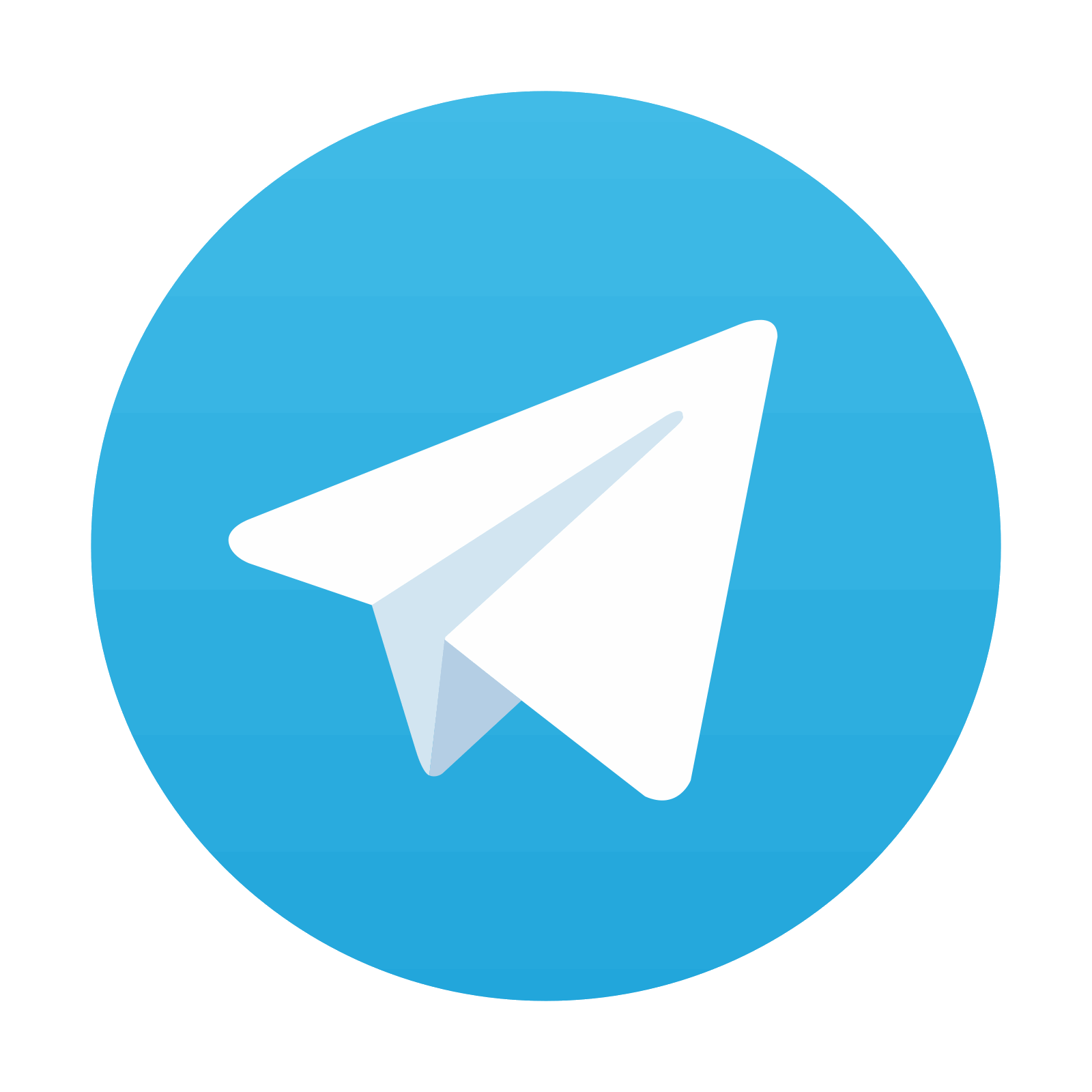
Stay updated, free articles. Join our Telegram channel
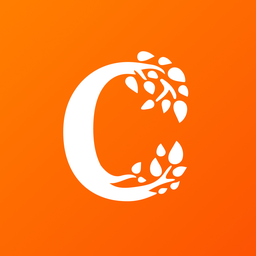
Full access? Get Clinical Tree
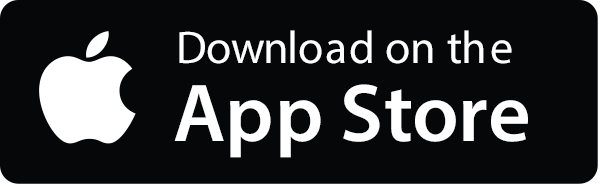
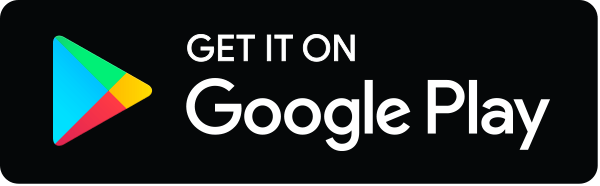