Fig. 10.1
Schematic drawing describing distinction between the receptor prosthetics and the somatic prosthetics. Firing of the receptors will send the brain about the current state of the body (sensory signal; top-left panel). Receptor prosthetics will substitute/simulate the receptor firing; thus, the reorganisation of the neural representation of the body will not be required (bottom-left panel). In contrast, sensorimotor processing requires transformation of sensory signal to the motor output (top-right panel). Therefore, the somatic prosthetics will require reorganisation of the neural representation of the body, depending of the quality of the novel receptor signal (bottom-right panel)
We dwell on this distinction in some detail, because it does useful scientific work. In particular, it clarifies the distinction between afferent signals and body representations. A replacement receptor such as a cochlear implant mimics afferent signals, but does not necessarily change body representations. In contrast, rewiring the topology of wiring between the brain and the body implies a change in the brain’s representation of the body, i.e. a change in the central map of the sources of afferent signals from the periphery and of destinations of efferent signals to the periphery. In the neuroscientific literature, the term ‘body representation’ has generated considerable confusion. We believe that considering these two styles of prosthetics can provide an important operational definition of body representation. Therefore, in this chapter, we will not be concerned with the neural coding of individual afferent signals nor with how those signals can be mimicked prosthetically. Rather, we will consider how the brain creates and maintains a central map of signal sources and destinations. This map can be considered a representation of what the body is like, i.e. of its physical organisation and its capacity to interact with the world. It is this form of body representation that primarily interests work in somatic prosthetics.
The relation between primary signals and body representations is an interesting and complex one. The two levels are clearly related, because we have defined body representation as organised maps of signal routing. We suggest that body representations arise by a process of synthesis and integration from statistical patterns of firing across multiple signals. For example, by monitoring the afferent activity that follows firing in each of two primary efferent signal pathways, the brain might learn an ordinal spatial relation between the two afferent and two efferent signals. This process is sufficient for emergence of a map of the corresponding body regions where the afferent and efferent signals project [1, 2]. Thus, for an organism to have a body representation, it must, at least implicitly, have the capacity to detect and monitor correlations across multiple parallel signals within a common projection pathway.
Our first question is therefore whether body representations, defined in this way, actually exist in the brain. We will use evidence from skilled motor control to suggest that they do exist, at least at an implicit level. Second, show that these representations depend on the capacity to integrate multiple signals to produce a synthetic percept and that this integration takes account of the relations between the various contributing signals. To do this, we argue from the special case of the perceived position of the hand in space. Third, we examine the systematic distortions often found in explicit body representation tasks. These distortions suggest that the synthetic representation of the body is only imperfectly separated and abstracted from underlying sensory signals. We next consider the processes that lead to the change in the body representation. We will argue for the existence of two different mechanisms that can modify the perception of the body at different timescales. Understanding the different processes that modify body representations could provide a useful way to study the content of the body representations themselves.
In the final section we discuss specific questions related to neuroprosthetic applications. In particular, we investigate how neuroprosthetics use can change body representations, and we make some suggestions for the future direction of this research.
10.2 Body Representations and Sensorimotor Control
A first important question is whether such body representations exist within the human mind or brain. Put another way, can behaviour be explained simply by arcs between individual afferent and efferent signals, or must we posit some central representation of the sensory and motor body?
Aimed movements to reach for a target are a fundamental form of sensorimotor coordination. In computational models of motor control, a representation of the target in external space is used as input to a planner which selects appropriate joint angles (the inverse kinematics transform) and torques to achieve them (inverse dynamics). Importantly, the inverse kinematics transform requires information about the lengths of different limb segments in order to compute appropriate joint angles. Therefore, even simple skilled movements presuppose both signals coding joint angles and some representations, at least implicit, of body size.
Here, we consider the specific case of proprioceptive or position sense. For example, many sensory receptors contribute to knowledge about the positions of body parts and the configuration of the limbs. Muscle spindle afferents probably play the dominant role in signalling limb configuration, but joint receptors, tendon organs, cutaneous afferents and central signals may all also contribute. Most work on proprioception has focused on perceiving the angle of a single joint on the basis of spindle discharge from a single muscle [3]. However, people are able to perceive the position of the hand in space without vision, suggesting that proprioceptive information from several muscles is propagated along the kinematic chain of the limb to compute endpoint position [4].
Interestingly, tasks requiring explicit proprioceptive judgement of hand position showed evidence of several levels of integration and synthesis. First, the mind synthesises an awareness of the hand position as distributed continuously throughout external space [5]. This spatial continuity is remarkable, given that the underlying afferent signals originate from highly discontinuous receptors, distributed in several separate muscles acting around the shoulder and elbow, and given that the precision of muscle afferents remain the fundamental limitation in accurate perception of hand position [6, 7]. The perceived position of the hand shows two characteristic distortions, namely a lateral shift towards the shoulder of origin and a rotation that produces proximal errors ipsilaterally and distal errors contralaterally. This pattern implies that hand position initially is represented in a reference frame, or ‘propriocentre’ based on the shoulder, rather than the head or torso.
In addition, a second level of synthesis integrates proprioceptive information from the two arms, referring hand position to a common egocentre. For example, we found that expert dancers show smaller lateral and rotational shifts in hand perception than nondancers. That is, the perceived position of the hand in space depends on which hand is being judged, but this dependence is reduced in proprioceptive ‘experts’. We may hypothesise that the proprioceptively derived representations of each hand are more completely integrated into a coherent representation of the body as a whole in the case of the dancers. Of course, we cannot be certain whether this difference reflects either a result of extensive sensorimotor training or a predisposing factor for becoming an expert dancer. For current purposes, the important point is that afferent signals are not only processed in a local, piecemeal fashion but are integrated into a coherent representation of the body.
10.3 Afferent Signals and Supra-afferent Information in Body Representation
We have already mentioned that the computations underlying simple reaching movement require information about both the current angles of the joints and the lengths of limb segments. Information related to joint angles arises from several classes of peripheral receptors. However, no sensory receptors directly signal the size of body parts. On this basis, we suggest that the representation of the body requires integration of two quite different kinds of information: current afferent signals and ‘supra-afferent’ information about the physical feature of the body, which is not directly signalled. In this section, we consider the nature and origin of this ‘supra-afferent’ information and how it contributes to body representation.
Consider again the length of a body part: for example, how do we know the size of our index finger? Intuitively, we may look at our hand to establish this. If vision of the hand is occluded, we may rely on stored information. Critically, there is no single, or even combined, somatosensory signal that can supply the relevant value. I could haptically explore my left index finger with my right index finger and use the proprioceptively sensed change in the right metacarpophalangeal joint to compute the distance that the right fingertip has to travel to stroke the left index along its length. However, this computation involves the forward kinematics of the right index finger, which in turn requires information about finger length—petitio principii.
Longo et al. [8] recently investigated the mental representation of body part size using an implicit measure based on position sense. Participants were asked to indicate the locations of the tips, or knuckle of each finger, by pointing with a stick on a board placed above the hand. Importantly, each judgement was made independently on a blank field, so there was no explicit requirement to represent the size of body parts, including the lengths of the fingers. Instead, the mental representation of finger length was calculated implicitly by comparing the perceived positions of the finger and knuckle of each finger. The results showed large and systematic distortions in the perception of body part size. In essence, people represented their hands as shorter and fatter than they really were, compressing the lengths of the digits and increasing the spacing between the knuckles. In contrast, when people were asked to pick a picture of their hand from a range of photographs that had been distorted to have different aspect ratios, they performed almost perfectly. Thus, recognition of spatial metric body properties appears to be highly accurate, yet production of the same metric properties on the basis of body representation appears highly distorted.
Interestingly, similar distortions were found for other body parts. Fuentes et al. [9] developed a body image task (BIT) in order to measure the coherence and relative proportion of body representation, as originally proposed by Daurat-Hemilijak et al. [10]. Participants viewed a screen on which a small outline drawing of an ‘anchor’ body part, such as the head, was presented at a random location. They then saw the name of a given bodily feature (e.g., ‘left shoulder’). This instructed them to click at the screen location where that feature would be on their body, given the location and scale of the anchor body part visible on the screen. By asking people to indicate the locations of several body parts relative to the anchor, the representation of the entire body could be implicitly reproduced. An analogous face image task (FIT) was used to measure the represented structure of the face, by pointing to the locations of features such as eyes, ears and chin, relative to an anchor point on the tip of the nose [11]. Whereas the hand representation was based on direct proprioceptive matching of different features, the BIT body image and FIT face image used a less direct method, involving a transformation of information about the body into a visual representation on the screen. The body and face images are therefore explicitly based on a stored representation of the position of body features relative to an anchor point, while the hand task is not.
Nevertheless, all three tasks revealed the same distortions of perceived shape, with a tendency to underestimate height and overestimate width (see Fig. 10.2). One might say that the mental representation of the body is pushed away from the neuraxis in an orthogonal direction and also fails to expand along the direction of the neuraxis itself. This pattern shows that a metric spatial representation of the body exists, that it contributes to both current perception of the body and stored knowledge about the body and that it exhibits a general anisotropic distortion. Since these distortions are quite large, they would presumably lead to large errors in aimed movement if the same body representation were used both as a mnestic representation of body structure and also for online motor control. Therefore, it seems likely that the information about relative positions of body landmarks is stored separately from the information about body segment lengths required for kinematic transformations.
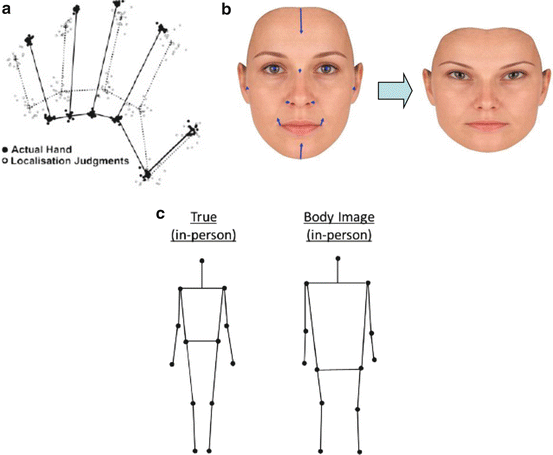
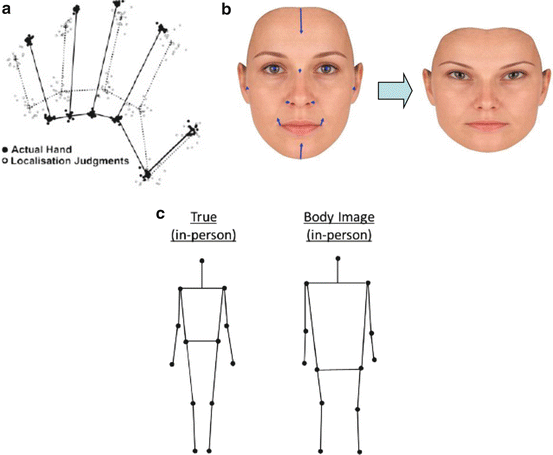
Fig. 10.2
Common shape distortion in the mental representation of (a) hands, (b) faces and (c) bodies. (a) Filled dots with solid line: average actual knuckle and fingertip positions. Open dots and lines: perceived knuckle and fingertip positions and implied hand representation. From Longo and Haggard [8] with permission. (b) Errors in represented positions of facial landmarks relative to an anchor on the tip of the nose and morphed image showing implied face shape. From Fuentes et al. [11] with permission. (c) Actual positions of body landmarks (black dots) and represented positions (open circles) relative to an anchoring head image. From Fuentes et al. [9] with permission
Why is the representation of the body distorted in this way? Can these distortions offer any clue regarding its origin in the brain? Further research on this point is needed, but several pieces of circumstantial evidence link these distortions, and thus the mental body representation itself, to the somatosensory system. Since the representation of the hand has been the most extensively studied, we focus on the hand: future research will be required to investigate whether these comments can be generalised to other body parts. First, the hand representation anisotropy is the inverse of the anisotropy that characterises the receptive fields (RFs) of somatosensory cortical neurons. Neurons in primary somatosensory cortex with receptive fields on the fingers, and, to a lesser extent, on the palm, generally have elliptical receptive fields. The long axis of the RF stretches proximo-distally along the digit itself, while the short axis runs medio-laterally. The body representation could potentially be based on counting the number of receptive fields on the skin overlying a particular body part. Thus, the anisotropic nature of RFs means that few RFs are traversed between finger and knuckle, while several are traversed between the base of one finger and the next. Estimates of distance between body features based on simple counting of RFs would hence produce a shortened, widened hand image. However, this explanation requires the additional premise that the neural circuits computing body representation have access only to the number of RFs, and not to their spatial organisation. More positive evidence for a somatosensory basis of body representation comes from analysis of digit lengths within the hand representation. Longo et al. [8] used principal component analysis to investigate the pattern of variability across individuals in represented length across the five digits. They found stronger correlations between digits that lay within a single dermatome, compared to digits in different dermatomes. As a result, the principal components of the hand representation closely corresponded to the established dermatomal organisation of somatosensory afferents. As a general principle, we suggest that fixed patterns of distortions in body representation may reflect intrinsic constraints in the information that the brain receives from the body. Because body representation involves integrating the local receptor information to form the whole-body representation, these constraints remain present at more abstract levels of representation. For example, the anisotropic shape of tactile receptive fields may explain the distorted model of the hand (see Fig. 10.2).
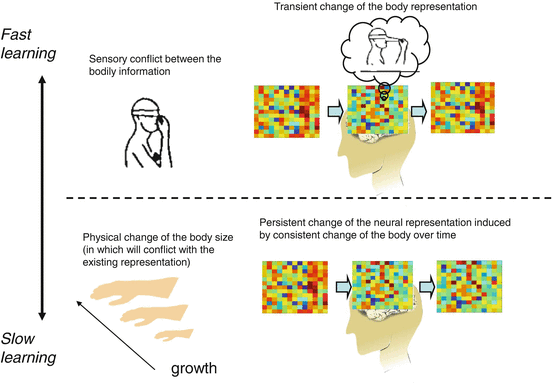
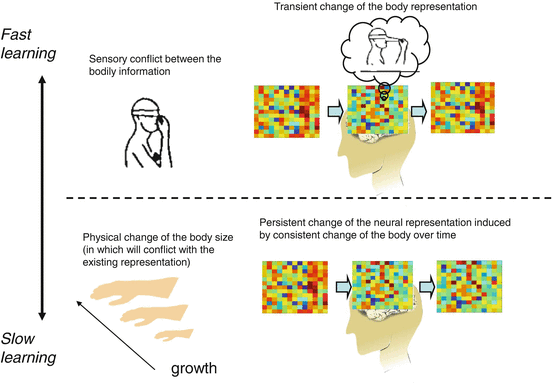
Fig. 10.3
Schematic drawing describing the fast and slow learning process for updating body representation. When there is a conflict between the sensory signals from the body, like movement sensation of the arm while the hand touching the nose [27], the brain creates a tentative solution of percept to maintain the coherence of the integrated body (i.e. nose elongation). This will also tentatively change the neural representation of the body, but the change will not be stable (upper panel). In contrast, if the conflicting situation is consistent over time, such as by due to the growth of the body, the neural representation of the body will be persistently changed
A question of intense interest for somatic neuroprosthetics is to understand how sensitive the body representation may be to altered patterns of sensory input. For example, would artificial sensory stimulation of particular digits lead to changes in hand representation, perhaps paralleling the known plastic changes in somatosensory cortex [12]? Altered visual input using virtual reality may also cause changes in body representation [13], but these are often only short lasting. Thus, the mental representation of the body may involve a core structure that is updated by current input. Interestingly, the same pattern of distortions was seen in the hand representation of a congenital phantom hand [14] as in the hand of two-handed volunteers. This raises the interesting possibility that an innate spatial organisation of somatosensory cortex may provide the basic structure of body representation. Future neuroprosthetic research could usefully examine whether and how prosthetic use can influence this basic structure.
10.4 Flexible Body Representation: Fast and Slow Process for the Plastic Change
In this section, we discuss the plasticity of the body representation: how flexibly can the perceived metrics of our body be changed? As discussed in the previous section, the brain seems to possess a model of the body. This model is created and updated through multisensory (visual, somatosensory, auditory) input regarding the body. However, any sensory information can be corrupted with noise. The brain must figure out which information should be used to update the body representation and which should not. One possible solution for the brain is to use the information about the temporal stability of the incoming sensory information. If a certain change of the body is stable over time, it may be the ‘true’ change. If not, the brain should ignore merely transient changes and should not update the body model. Similar computations may take place when the brain estimates whether the incoming sensory information is a consequence of one’s own body movement or not [15, 16]. However, the focus here is about the information already assigned as self-related.
In motor learning studies, it has been postulated that the brain combines multiple motor learning systems, which adapt to the environment at different timescales [17, 18]. For example, in the task of Kojima et al. [19], monkeys learned to increase the gain of the saccade to a certain point and then decrease back to the original gain. After this procedure, when the monkey was again asked to increase the saccade gain, the speed of learning was faster (‘savings’) compare to the first time the increased gain was encountered. Interestingly, even if there was a prolonged gap between the end of the gain decrease condition and the second gain increase condition, during which the monkey made saccades at the baseline gain in total darkness without visual feedback, the pattern of performance still showed the ‘saving’ pattern associated with prior learning. This phenomenon was explained by postulating two separable learning systems: a fast and a slow system [17, 18]. The systems are distinguished by the way that they handle error information. One system quickly adjusts the motor command according to the current error signal. This means that it learns fast, but forgets fast. The other system reflects the error information into the motor command more gradually. So the system significantly learns only if the situation is stable over time. As a result, this system will learn only slowly, but it also forgets only slowly. The final motor output of a given trial will reflect the output from both of the two systems. In the case of Kojima et al. [19], the slow system had not yet forgotten about the increased gain even after a gain decrease, producing the ‘saving’ phenomenon.
In analogy to these findings in the motor learning literature, we suggest that similar dual systems may exist in body representation [20]. In motor control, the error signal is the difference between the expected sensory consequence of action and the actual sensory input. For body representation, the error signal will be the difference between the stored model of the body and the current sensory input from the body. Likewise, the output from the learning system will not be the adjustment motor command to compensate for the error, but will be an internal signal that would maintain the coherence of the body representation under conditions of conflict or error—a form of somatosensory conflict resolution.
As in motor control, we may distinguish a fast and a slow system for updating body perception. The fast learning system would typically be involved in adjusting the relationship between the bodily information provided from different sensory channels. Since the reason for such adjustment is to cope with noisy, conflicting sensory information and since noise levels fluctuate rapidly with environmental and system conditions, the stored representation is fragile and will not last long. Indeed, this sort of plastic change must be quite short lived, so that the brain is ready for the next sensory perturbation.
The other system is a slow learning system. We suggest this has the major role of adjusting for physical change of the body, for example, during growth [18]. Physical change of the body occurs in a much slower timescale than the transient environmental change. Consequently, physical change is expected to be reflected on the perception of the body representation in a more slow timescale. At the same time, the updated representation must be robust, in a sense that it should not be easily affected by a sudden environmental change or a single input value. We suggest that the updating procedure of the slow system is done on the basis of whether the parameters of the fast system are stable over time. From the nature of slow learning system, only stable information will be reflected to update the representation. Below, we will discuss the evidences for this account.
We will not particularly focus on the semantic aspects of body representation. For example, in the following section, we will describe some illusions that induce distortions of the body shape. Importantly, participants will feel the distortion of the body (e.g. my nose is elongating), but at the same time, they know that their body is not actually distorted (the shape of my nose has not and will not change); from the abstract knowledge, they have about one’s body. Though the knowledge about one’s own body may reflect the accumulated history of the feeling of the body, here, we will only focus on the first-order perception (feeling aspect) about the body, and not on general semantic information about ‘what the body is really like’. See Longo et al. (2010) for the review about such structural knowledge about the body.
10.4.1 The Fast Plasticity System Adjusts Body Representation in Noisy/Conflicting Sensory Environments
We have seen that the brain can access information from and about the body via multiple sensory feature channels, both within the same modality and across different modalities [21]. For example, the brain can estimate the position of the right index finger from the local receptor firing of the wrist and shoulder muscle/joint or from the position of the left hand touching the right hand or by directly seeing the hand. However, since any sensory input can be affected by noise, there could be a potential conflict among the multiple sensory estimates. To maintain coherent body representation in such potentially conflicting situation, continuous adjustment between these inputs is necessary [22]. Experimental manipulation of somatosensory information has been a successful experimental tool to observe how the brain copes with the conflicting inputs and how it modifies the perception of one’s own body while solving the conflict. Some dramatic modifications of body representation have been reported in tendon vibration experiments.
When a tendon of a limb muscle is mechanically vibrated at the frequency of 80–100 Hz (e.g. wrist extensor muscle), people experience a sensation of a slow movement (e.g. wrist flexion) of their vibrated limb in the absence of actual movement, intention to move or sense of effort [23]. The vibration of the tendon excites the muscle spindle afferents that conveys muscle stretch information [3, 24–26]. Since the muscle stretch information is the major contributor for detecting limb displacements, the brain interprets this input as caused by a movement of the limb joint. In another word, the vibration can mimic the afferent activation of limb movement, and the brain creates the perception that follows what the neuronal activity usually tells us about. Therefore, this simple version of the vibration illusion will not change any perception about the base parameters (shape/size) of the body representation (by analogy with receptor prosthetics; see above). However, when the movement information induces a sensory conflict with other somatosensory information, this illusion starts to induce complicated perception about morphological changes of the body. These illusions are phenomenally compelling, even though we well know that the corresponding events would be very unusual in our daily life.
One famous example is reported by Lackner [27]. In his experiments, while the participants touched their own nose with their right index finger, the tendon of the biceps brachii (elbow flexion muscle) was vibrated. Participants felt the part of their nose touched by the finger elongating, following the illusory movement of the finger that accompanied the elbow flexion experience (Pinocchio illusion). What is the logic of the brain underlying this phenomenon? Here, two conflicting pieces of information coexist. One is the information of the finger movement induced by tendon vibration. However, the touch sensation on the nose tells the brain that the finger is not moving, since the nose is not moving. To make sense out of this situation, it seems that the brain prioritises the incoming movement information over the static information about the nose position and creates the percept that the nose moves (elongate) together with the finger movement. Similar changes in perceived metrics of the body were also reported by de Vignemont et al. [28] and Ehrsson et al. [29]. The former showed a perception of finger elongation during vibration when the finger is held by the opposite hand and the opposite elbow muscles are vibrated. The latter demonstrated the perception of waist shrinkage, when both left and right palms of the hands were touching the waist and the illusion of wrist flexion was simultaneously induced for both of the wrists. Longo et al. [30] demonstrated a slightly different variant of the body-shrinking illusion. They showed that the forearm length can be felt as shortened, when the tendon of the biceps brachii and the triceps brachii were both vibrated at the same time. The reason why this shortening happens is unclear, because it is not directly signalled by any of the receptors involved. However, as the authors suggest, the situation involves processing of conflict between the information from the two muscles, each sending extension and flexion movement of the elbow, respectively. Representing one’s own body as a spatially extended, volumetric object in external space may require a coherent representation in which such multisensory conflicts have been solved. In contrast, the presence of such multisensory conflicts may prevent the formation or maintenance of a coherent spatial field and may prevent the representation of the body as a spatially extended object within that field.
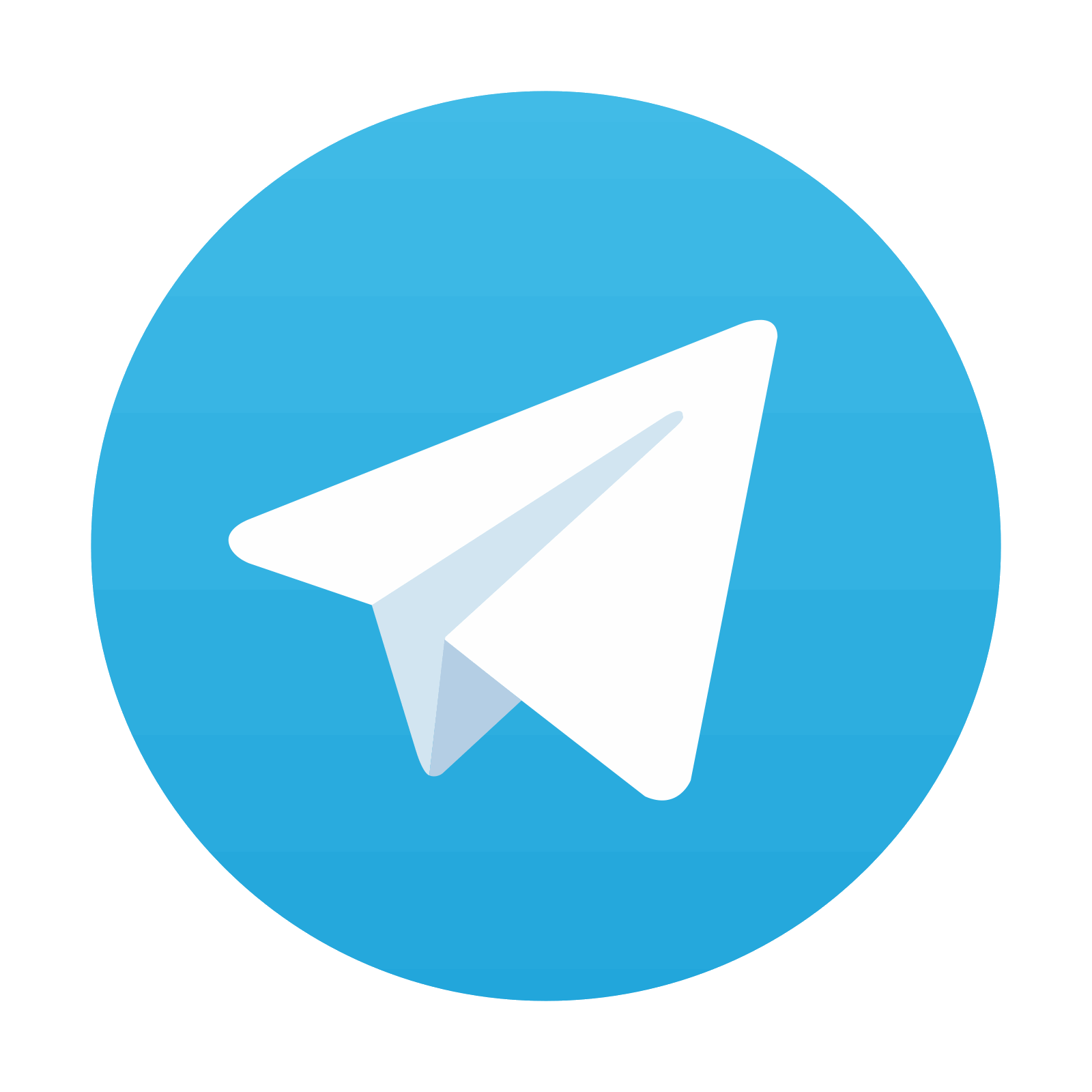
Stay updated, free articles. Join our Telegram channel
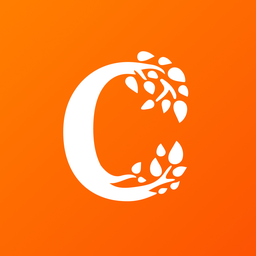
Full access? Get Clinical Tree
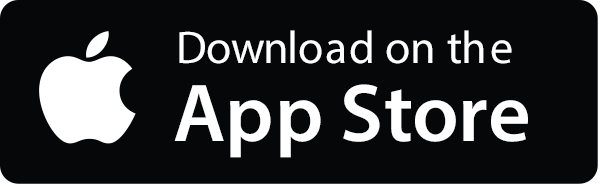
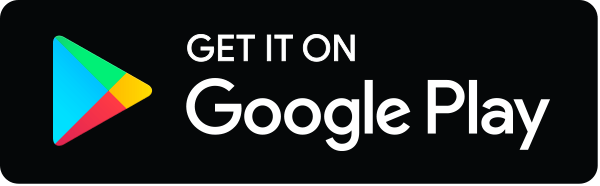