Fig. 4.1
BMSC therapy induces neurorestorative effects post stroke
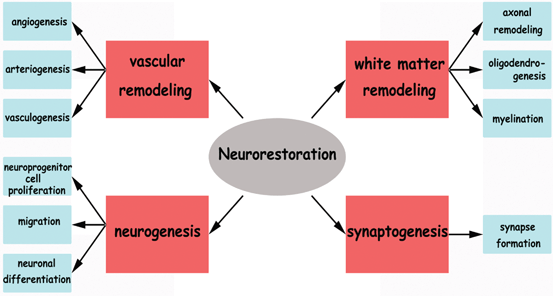
Fig. 4.2
The various aspects of neurorestoration that are targeted by cell therapy to improve neurological recovery post stroke
4.3.1 BMSC Migration into the Ischemic Brain
Many studies have confirmed the ability of BMSCs to migrate to the injury site (Lee et al. 2003; Wu et al. 2008). BMSC therapy initiated intravenously at 1 or 7 days after stroke in an animal stroke model promoted neurovascular remodeling resulting in improved neurological function post stroke (Chen et al. 2001a, 2003a; Shen et al. 2007). Administered BMSCs were found to survive and migrate to the ischemic brain. The disruption of the brain-blood-barrier (BBB) may contribute to the entry of BMSCs into ischemic brain compared to normal cerebral tissue thereby aiding BMSC migration to the ipsilateral hemisphere. BMSCs harvested from mouse were labeled using a nuclear fluorescence dye, bis-benzimide, for 24 h. The labeling of BMSCs enabled visualization of migration and differentiation of the implanted BMSCs . The labeled BMSCs were stereotactically transplanted into the ischemic striatum in mouse post MCAo (middle cerebral occlusion) model. Migration of the implanted BMSCs to the injured cortex and corpus callosum was observed. Four weeks post transplantation, some BMSCs were found to express neuronal markers like NeuN (neuron-specific nuclear protein) (Zhao et al. 2002). After 7 days of BMSC transplantation in animals that underwent transient MCAo and were treated at 24 h post stroke, migration of BMSCs to tissues supplied by the middle cerebral artery was observed (Wu et al. 2008). Another interesting study included transplantation of BMSCs derived from green fluorescent protein (GFP) expressing transgenic mice, 7 days post permanent MCAo in mice, followed by in vivo tracking of transplanted cells using fluorescence optical imaging (Shichinohe et al. 2004). The emitted green fluorescence was observed by exposing the skull and using in vivo fluorescence optical microscopy. Observations over the following 4–12 weeks revealed that the BMSCs migrated towards the ischemic injury site and immunohistochemistry analysis indicated accumulation in the ischemic border zone (IBZ) and some expressed neural phenotypes (Shen et al. 2007) .
4.3.2 BMSC Differentiation
BMSCs have the ability to differentiate into neural and mesodermal cell lines (Zhao et al. 2002) . BMSCs can stimulate nerve regeneration (Tohill et al. 2004). An in vitro study, in which BMSCs were co-cultured with fetal brain neural cells, suggests that differentiation of BMSCs to neurons can be promoted by close proximity to neural cells (Yan et al. 2005) . When BMSCs were cultured in the presence of growth factors like BDNF or EGF, they expressed glial fibrillary acidic protein (GFAP), NeuN, and nestin (a marker for neural precursors), suggesting the capability of BMSCs to differentiate into neuronal cells (Sanchez-Ramos et al. 2000). Purified human BMSCs were grafted into the cortex surrounding the area of infarction 1 week after stroke in rats, and some transplanted human BMSCs expressed markers for astrocytes, oligodendroglia, and neurons (Zhao et al. 2002). However, the morphological features of the grafted cells were spherical in nature with few processes and did not show neuronal function (Zhao et al. 2002). In addition, treatment with BMSCs via intravenous administration demonstrated that very few BMSCs differentiated into neurons or astrocytes in the ischemic brain in vivo (Weaver et al. 1991; Chen et al. 2001a, 2003a; Shen et al. 2007; Li et al. 2008). Although it has been suggested that differentiation of BMSCs into cells of neural lineage may occur both in vitro and in vivo, BMSCs do not survive long term and replace damaged tissue (van Velthoven et al. 2012) . Therefore, it is highly unlikely that the functional recovery observed by the ischemic rats with BMSC grafts was mediated by the integration of new “neuronal” cells into the circuitry of the host brain (Chen et al. 2001a; Zhao et al. 2002).
Only a small fraction of the injected BMSCs expressed neural phenotype and the mechanism of action of the therapeutic effects of BMSCs differ from that originally proposed for stem cells therapy. Stem cells being totipotent may replace injured/dead brain cells. The stem-cell like population in the BMSCs function in a similar manner and may differentiate into neural cell lines (Zhang et al. 2009; Shichinohe et al. 2010; Ding et al. 2011). However, these cells are a minor subpopulation of the BMSCs and do not contribute to the restoration of function, and only a very small percentage of the BMSCs assume parenchymal cell phenotype (Li et al. 2002). The neurological benefit induced by BMSC treatment appears to be derived primarily from the release of growth and trophic factors (van Velthoven et al. 2012) .
4.3.3 BMSCs Secrete Trophic Factors
Exogenous BMSCs have been shown to produce, and more importantly, to stimulate the production of neuroprotective and neurorestorative factors in parenchymal cells. A broad range of mRNAs for angiogenic and arteriogenic cytokines are expressed by the BMSCs. Some of these are vascular endothelial growth factor (VEGF), basic fibroblast growth factor (FGF2), placental growth factor, insulin-like growth factor and Angiopoietin-1 (Ang1) (Chen et al. 2003b; Zacharek et al. 2007). Human BMSCs when cultured in the supernatant derived from ischemic rat brain extracts increase their production of several growth factors like brain derived neurotrophic factor (BDNF), VEGF and hepatocyte growth factor (HGF) (Chen et al. 2002a, b). The release of these growth factors contributes to neurological recovery by initiating cell repair mechanisms, increasing cell proliferation in the sub-ventricular zone (SVZ) and reducing apoptosis in the ischemic region (Li et al. 2002) and thereby improve functional benefit (Chen et al. 2002a, b, 2003b; Zacharek et al. 2007; Wakabayashi et al. 2010).
bFGF protects brain cells against toxins and promotes cell survival. Following focal cerebral ischemia in animal models, intravenous bFGF administration can reduce infarct volume and stop the down-regulation of anti-apoptotic protein Bcl-2 (Ay et al. 2001). bFGF therapy has neuroprotective effects when administered within a few hours after ischemic onset and reduces infarct volume; and neurorestorative effects when administered a day after the ischemic attack by enhancing synaptogenesis and neuronal sprouting (Ay et al. 1999). BDNF is associated with neuronal survival, learning and memory, neuroplasticity and synaptic plasticity. Increase in BDNF has been correlated with improved behavioral recovery following brain injury. In an animal stroke model, BDNF showed therapeutic promise for functional recovery post stroke (Ploughman et al. 2009). Glial cell-derived neurotrophic factor (GDNF) promotes endogenous cell repair mechanisms, increases neurogenesis, neuroblast proliferation and migration from the SVZ and decreases apoptosis (Kobayashi et al. 2006; Yuan et al. 2013). Nerve growth factor (NGF) promotes cortical neurons against ischemic insults. The NGF/receptor system has been suggested to play an important role between astrocyte/neuron interaction under focal cerebral ischemia (Lee et al. 1998). Decrease in delayed death of hippocampal neurons post ischemia has also been reported upon treatment with NGF (Lee et al. 1998). VEGF promotes angiogenesis and is also capable of exerting neuroprotective effects (Sondell et al. 1999). VEGF decreases post ischemic lesion volume, apoptosis, and overall neuronal damage (Hayashi et al. 1998). Hence, enhancing trophic factor and growth factor production may be the mechanism of action in BMSC therapy. BMSC treatment increases the release of neurotrophins and angiogenic growth factors like bFGF, BDNF, GDNF, HGF, NGF, VEGF, etc. from parenchymal cells and thereby promotes endogenous brain repair mechanisms (Chen et al. 2001a; Hardy et al. 2008).
The intravenously administered BMSCs migrate to the brain and stimulate the endogenous cells (mainly astrocytes and endothelial cells) to produce growth factors, which in turn promote angiogenesis and vascular stabilization, partially mediated by VEGF/Flk1 and Ang1/Tie2 pathways (Zacharek et al. 2007). Insulin-like growth factor 1 (IGF-1) is a natural activator of the AKT signaling pathway. It is also known to stimulate cellular growth and proliferation and inhibit apoptosis. When cells were subjected to ischemic insult, elevated IGF-1 mRNA expression levels were observed (Wakabayashi et al. 2010). Upon BMSC therapy, enhanced expression levels of IGF-1 mRNA, IGF-1 and IGF-1R immuno-reactive cells was observed, suggesting that the neurological functional benefit may also be derived from an IGF-1 mediated self repair mechanism (Wakabayashi et al. 2010).
4.3.4 BMSC Treatment of Stroke Induces Neurorestorative Effects and Increases Interaction/Coupling Among Restorative Events
The conceptual model of the neurovascular unit focuses on illustrating the dynamic interactions among the brain neurons, micro-vascular endothelium, extracellular matrix, astrocytes and pericytes. While the neurovascular unit has gained importance as a key component of stroke damage, another model called the vascular neural network, encompassing the neurovascular unit and the vasculature supplying and interacting with the neural structures of the brain, is gaining attention. Stroke can inflict extensive damage to the vasculature of the brain along with damage to the neural circuit. Hence, the vascular neural network is a potential target for neurorestorative therapies (Zhang et al. 2012). The vascular neural network is a collection of cell types and structures inclusive of capillary endothelial cells, astrocytes , pericytes and neurons that constitute the neurovascular unit and structures connecting with arteries and arterioles upstream of the cerebral microcirculation, like vascular smooth muscle cells, arterial endothelial cells, perivascular nerves and venules downstream (Zhang et al. 2012). The vascular neural network can potentially target all five therapeutic aspects of stroke recovery; retardation of injury, reperfusion of ischemic penumbra, reduction of tissue injury, regeneration of new cells and reorganization of vasculature and neural networks (Zhang et al. 2012). Following brain injury, similar to how cortical gray matter recovery is mediated by a neurovascular niche, white matter recovery may be mediated by an oligovascular niche that promotes oligodendrogenesis and angiogenesis (Pham et al. 2012). These concepts are evolving with accumulating literature and influencing the neurorestorative approach towards stroke recovery.
Angiogenesis and neurogenesis in the neurovascular niches of the CNS play a key role in recovery. Neurogenesis and angiogenesis are linked together by growth factors and chemokines and trophic effects of Stromal cell-derived factor 1 (SDF1) and Ang1 which are up-regulated by blood vessels within the niche, and improve behavioral recovery (Ohab et al. 2006). Results from an animal stroke model indicated improved post stroke neurological recovery that was partly attributed to the migration of newly born neurons in the SVZ to the cortex, neurogenesis derived from GFAP-expressing progenitor cells in the SVZ, and migration of neuroblasts to a neurovascular niche in peri-infarct cortex (Ohab et al. 2006).
BMSC treatment of stroke induces expression of many angiogenic and trophic factors, and thereby regulates interaction/coupling between restorative events. The neurorestorative events include: vascular remodeling, white matter remodeling , neurogenesis and synaptic plasticity which may collectively contribute towards BMSC treatment induced neurorestoration after stroke .
4.3.4.1 BMSC Treatment of Stroke Increases Vascular Remodeling
Vascular remodeling includes angiogenesis and arteriogenesis and plays a major role in regulating cerebral blood flow (CBF). Cerebral blood flow regulation and the re-establishment of functional microvasculature via angiogenesis and arteriogenesis in the IBZ helps maintain neural function and creates a hospitable microenvironment for neuronal plasticity leading to functional recovery (Plate 1999; Chen et al. 2003b; Pratt et al. 2004). Enhanced vascular remodeling improves blood supply to ischemic cells and mediates the generation of restorative trophic factors. Vascular remodeling leads to the formation of mature and functional blood vessels and hence has gained much importance as a therapeutic target.
Angiogenesis, defined as the process of growth of new blood vessels from pre-existing vessels, has been associated with improved long-term recovery of stroke patients (Arenillas et al. 2007; Navarro-Sobrino et al. 2011). Tissue ischemia and hypoxia stimulate angiogenesis hours after the ischemic attack and angiogenesis may continue for weeks (Dor and Keshet 1997). Several growth factors like VEGF, endothelial nitric oxide synthase (eNOS), bFGF, platelet derived growth factor (PDGF) and Ang-1 mediate and tightly regulate angiogenesis (Greenberg 1998). The physiological sequence of angiogenesis that occurs in the penumbra of the ischemic brain is as follows. Angiogenic growth factors bind to specific receptors located on the brain endothelial cells and stimulate endothelial cell proliferation, migration and sprouting from the existing vessel towards ischemic brain area (Greenberg 1998). The first step in this process is vasodilation initiated by NO (Carmeliet 2000) which in combination with the increase in VEGF expression increases vascular permeability allowing extravasation of plasma proteins that lay down a provisional scaffold for the migration of endothelial cells for vascular sprouting. Next, the sprouting endothelial cells form blood vessel tube-like structures. The initial vascular plexus forms mature vessels by sprouting, branching, pruning and promoting differential growth of endothelial cells, and recruitment of supporting cells, such as pericytes and smooth muscle cells (Folkman and D’Amore 1996; Risau 1997). This step involves the dissociation of smooth muscle cells and loosening the extracellular matrix which enwraps the mature vessel. Once the path of sprouting has been established, proliferating endothelial cells migrate to distant sites. Angiopoietin (Ang) -2, an inhibitor of Ang1/Tie2 signaling, may be involved in facilitating the detachment of pericyte cells from endothelial cells, while the matrix metalloproteinase (MMP) family of proteinases degrade matrix molecules and further weaken the vessel integrity (Feng et al. 2009). Once new blood vessel networks are formed, Ang1 which activates Tie2 receptors helps to stabilize networks initiated by VEGF. The Ang1/Tie2 interaction promotes pericyte recursion and mediates maturation of neovessels into more complex and functional vasculature.
Arteriogenesis is another process of restoring blood supply to an ischemic region when the main supply artery is blocked. Collateral vessels develop from pre-existing arterioles that adapt to supply the same end tissue as the blocked artery. While angiogenesis and arteriogenesis share some common features, they have quite distinctive pathways. Vessel collateralization is initiated by fluid shear stress due to pressure differences between perfusion territories (Erdo and Buschmann 2007). The circulating monocytes attract, adhere to the endothelium and invade to pave way for the collateral vessel development (Schaper and Buschmann 1999). These monocytes also produce growth factors like VEGF and proteolytic enzymes that enable migration and division of smooth muscle cells (Scholz et al. 2001). The smooth muscle cells coat the pre-existing capillaries transforming them into vessels with larger diameters (Buschmann and Schaper 2000; van Royen et al. 2001). Arteriogenesis and collateral vessel growth have also gained interest as therapeutic targets.
Studies indicate that BMSCs can participate in angiogenesis and increase arteriogenesis (Kinnaird et al. 2004; Zhu et al. 2011). Having a perivascular/vascular location, BMSCs contribute to vascular formation and function and might be harnessed to regenerate a blood supply to injured tissues (Watt et al. 2013). In vitro, BMSCs have also been reported to play an important role in angiogenesis in a time and dose dependent manner affecting all stages of angiogenesis from formation to maturation of vessels (Duffy et al. 2009). BMSCs implanted intravenously at 24 h post transient MCAo, expressed increased angiogenic factors, such as VEGF/FLK1 and Angiopoietin-1/Tie2 in the IBZ associated with enhanced functional of angiogenesis, vessel density and arteriogenesis as well as improved neurological functional recovery (Chen et al. 2003b; Zacharek et al. 2007; Wu et al. 2008; Cui et al. 2009). In a chronic limb ischemia model, treatment with autologous BMSCs significantly increased VEGF expression and increased vessel density (Hoffmann et al. 2010), improved blood flow and enhanced arteriogenesis (Zhu et al. 2011). BMSCs aid in brain repair by inducing a neovascular response by selectively targeting the injured tissue and improving blood flow to the ischemic zone via arteriogenesis and angiogenesis (Cui et al. 2009). These angiogenic and arteriogenic vessels are stimulated to produce trophic and growth factors that in turn contribute to brain plasticity and recovery of post stroke neurological function (Kinnaird et al. 2004). These results suggest that BMSCs induce and promote angiogenesis and arteriogenesis in the ischemic brain tissue to improve blood supply and alleviate neurological deficits.
4.3.4.2 BMSC Treatment of Stroke Increases White Matter Remodeling
White matter remodeling includes oligodendrogenesis, myelination and axonal regeneration. Oligodendrocyte progenitor cells (OPC’s) produced in the SVZ differentiate to form oligodendrocytes (OLs). While OPC’s do not have myelin forming ability, the mature oligodendrocytes do. In the ischemic brain, these mature oligodendrocytes may restore the myelin sheath lost during the ischemic attack and also myelinate the newly formed/sprouted axons. Hence, oligodendrogenesis is critical to restore and improve axonal function and neuronal communication (Zhang et al. 2013).
Oligodendrogenesis plays a key role in remyelination of axons and neural communication. Oligodendrocytes are at a higher risk of damage from ischemia since white matter has limited blood supply when compared to gray matter, and there is very little collateral blood flow in deep white matter (Back et al. 2002). Loss of myelin is of primary concern caused by oligodendrocyte damage, as injured oligodendrocytes can no longer produce myelin. Detrimental inflammatory responses, like increased MMP-9 and MMP-2 expression have been reported to further worsen white matter damage (Chen et al. 2011a). Following an ischemic stimulus, a greater fraction of the neural stem cells in the SVZ form OPC’s which in turn differentiate into mature myelin producing oligodendrocytes in the corpus callosum or striatum in the brain. This is central to the repair damaged axons as well as to coating the newly sprouting axons with myelin. Myelin is a dielectric by nature with electrical insulating properties. The myelin sheath on the axons is not continuous and exposes the axon only at the nodes of Ranvier. This facilitates the much faster saltatory conduction over continuous wave conduction as the electrical impulses jump from one node to the next. There is mounting evidence associating increased OPCs and oligodendrocytes expression with improved neurological function post stroke in animal models.
Ischemia subjects axons to extensive damage impairing neuronal communication, and poor axonal regeneration and neuroplasticity have been associated with poor functional recovery (Walmsley and Mir 2007). Axonal remodeling begins 2–3 weeks post stroke and has been detected even at 28 days post the ischemic attack (Liu et al. 2009, 2010). Promoting axonal regeneration improves brain plasticity and can restore previously lost functions (Papadopoulos et al. 2006). Successful axonal remodeling is central to promoting brain repair and nerve regeneration.
White matter remodeling is enhanced by intra-carotid BMSC transplantation which is facilitated by axonal sprouting and re-myelination in the cortical IBZ and corpus callosum (Shen et al. 2006). BMSC implantation into the ischemic brain significantly reduced the transient MCAo-induced cortical loss and thinning of the white matter and enhanced cortical beta-III-tubulin immunoreactivity (Chen et al. 2008). Significantly increased thickness of individual axons and myelin, and areas of the corpus callosum and the numbers of white matter bundles in the striatum also were detected in the IBZ of BMSCs-treated rats compared to stroked rats (Li et al. 2006). BMSC therapy also improved structural neuroplasticity and increased axonal outgrowth from healthy brain tissue (Andrews et al. 2008). BMSCs injected into the lateral ventricle of rats at 1 day post permanent MCAo improved neurological functional recovery by increasing synaptogenesis indicated by an increase in Synaptophysin expression in the IBZ (Weng et al. 2008). Intravenous administration of combination of BMSC with Niaspan in type-one diabetes mellitus (T1DM) stroke rats significantly increases myelin and axonal density in the striatum of white matter of the IBZ, as well as increases Synaptophysin and SMI-31 (a pan-axonal neurofilament marker) expression in the ischemic brain compared to T1DM-MCAo control (Ye et al. 2013). BMSC treatment of stroke also increases the number of oligodendrocyte precursor cells in the corpus callosum and enhances the areas of the corpus callosum in both hemispheres (Shen et al. 2006). These results suggest that BMSCs facilitate axonal sprouting and remyelination in the cortical IBZ and corpus callosum, which may underlie neurological functional improvement caused by BMSC treatment.
Corticospinal tract fibers originating from the contralesional motor cortex sprout into the denervated spinal cord after stroke and play an important role in motor functional outcome after stroke. BMSCs treatment significantly increases biotinylated dextran amine-labeled contralesional axons sprouted into the denervated spinal cord (Liu et al. 2008). The interaction of axonal remodeling between the bilateral-hemisphere also regulates functional outcome after stroke. Stroke induces spontaneous inter- and intra-cortical axonal plasticity originating from the ipsilesional motor cortex in adult rats. BMSC treatment of stroke enhances axonal plasticity and bilateral-hemisphere axonal remodeling (Liu et al. 2010). The axonal remodeling is significantly correlated with spontaneous functional recovery after stroke and recovery is significantly enhanced by BMSCs (Liu et al. 2010).
4.3.4.3 BMSC Treatment of Stroke Increases Neurogenesis
Neurogenesis is the generation of neurons from neural stem and progenitor cells primarily occurring in the sub ventricular zone (SVZ) and sub granular zone (SGZ) of the hippocampus . Post ischemic neurogenesis replaces damaged neurons and restores neuronal communication. Cell apoptosis and cell proliferation are tightly regulated and maintain the progenitor cell population (Shehadah et al. 2010). However, in a rat stroke model it was observed that following an ischemic attack, neurogenesis is increased and the greatly expanded neural progenitor cells migrate to the ischemic boundary regions and some differentiate into mature neurons (Parent et al. 2002). Based on an animal model of stroke, it has been reported that 48 h after stroke, the neural progenitor cell expansion in the SVZ is increased by the following modifications in the cell cycle (Zhang et al. 2006b). The cell cycle length is shortened from 19 h in non stroke cells to 15.3 h in stroke cells by reducing the duration of the G1 phase. A larger number of SVZ cells from the stroke group thus, re-enter the cell cycle after mitosis. Hence, the neurorestorative sequence is tightly controlled, starting with neuronal progenitor cell proliferation leading up to their differentiation into mature neurons. Thus, BMSC treatment of stroke not only regulates vascular and white matter remodeling , but also increases neurogenesis (Chen et al. 2003a; Zhang et al. 2004; Li et al. 2008; Yoo et al. 2008; Bao et al. 2011). Increased neurogenesis was observed associated with increased cellular proliferation in the SGZ and SVZ, and migration of NPCs to the IBZ and their differentiation into mature neurons. BMSC therapy increases neurogenesis, and migration and differentiation of neural cells, and also decreases apoptosis, as well as promotes neurological recovery (Yoo et al. 2008; Bao et al. 2011).
4.3.4.4 BMSC Treatment of Stroke Increases Synaptogenesis
The process of formation of new synapses is called synaptogenesis. Synaptic plasticity involves strengthening of synapses, via which, neurons communicate, and remodeling of axons, dendrites, etc. which in concert, improve brain functionality. Neurons communicate with other neurons and cells via the synapse by passing neurotransmitters across the synaptic cleft or communicating through electrical signals across gap junctions. Significant increase in synaptogenesis and improved functional outcome have been reported using several cell-based therapies, including intravenous or intracarotid artery BMSC treatment of stroke (Shen et al. 2006; Zhang et al. 2006a; Hayase et al. 2009; Ding et al. 2011, 2013; Gutierrez-Fernandez et al. 2013). This enhanced synaptogenesis was correlated with improvement in functional recovery (Gutierrez-Fernandez et al. 2013). Enhancing synaptogenesis improves neuronal communication and is an important target for neurorestorative therapy. The mechanism of BMSC treatment induced synaptogenesis may be related with BMSC secretion or stimulation of endogenous sonic hedgehog (Shh), endogenous tPA, NGF and Noggin expression (Shen et al. 2006; Ding et al. 2011; Shen et al. 2011; Ding et al. 2013).
4.3.5 Preconditioning of BMSCs and Treatment of Stroke
Hypoxia preconditioning is a strategy that enhances cell survival and apoptotic tolerance of neural progenitors and BMSCs in the ischemic brain (Theus et al. 2008; Francis and Wei 2010; Wei et al. 2012) . In adult rats, transplantation of BMSCs pre-treated with sub-lethal hypoxia (0.5 % oxygen) at 24 h after MCAo, had several benefits including increased angiogenesis and neurogenesis, decreased inflammation by inhibition of pro-inflammatory cytokines, decreased microglia activity in the brain and enhanced trophic factor production, all of which reinforced the benefits of BMSC therapy (Wei et al. 2012). Rapid and efficient functional recovery derived from similar beneficial effects and neuronal differentiation of neural progenitors from embryonic stem cells , resulted when hypoxia pre-conditioned embryonic stem cells were administered to ischemic rats (Theus et al. 2008). Treatment of stroke with BMSCs derived from stroke rats (Isch-BMSCs) also exhibited better functional outcome compared with BMSCs derived from normal rats (nor-BMSCs)(Zacharek et al. 2010). The enhanced beneficial effects may be related with the Isch-BMSC increased expression of Ang1/Tie2, VEGF/Flk1, bFGF, and GDNF expression and enhances angiogenesis, arterial density, and axonal regeneration compared with Nor-BMSC (Zacharek et al. 2010) .
4.3.6 Adverse Effect of BMSC Treatment in Diabetes Population
The benefits of BMSC therapy in stroke have been well established, but when BMSC therapy was extended to diabetic subjects, adverse effects were reported (Chen et al. 2011b) . When type 1 diabetic rats were subjected to stroke using the 2 h transient middle cerebral artery occlusion model and treated with BMSCs at 24 h post MCAo, no functional benefit was observed On the contrary, adverse reactions, including increased mortality, elevated BBB leakage, hemorrhage and vascular damage were observed. Elevated macrophage expression (indicated by ED1 marker) and angiogenin expression were associated with these detrimental effects. Clearly a treatment that works well in normal subjects did not translate to diabetic stroke subjects. In addition, BMSC treatment in T1DM stroke rats also increases arteriosclerosis-like vascular changes (Chen et al. 2011b), which may increase the likelihood of secondary stroke. Therefore, caution should be exercised while employing BMSCs treatment in diabetic stroke patients and the long term vascular changes should be investigated .
4.3.7 Combination BMSCs with Pharmacological Therapy
Another promising cell therapy strategy is the use of combination therapy . The strength of combination therapy lies in its ability to additively combine the positive effects of multiple treatments and at the same time overcome any adverse effects of one treatment. Combination therapy of Niaspan, an extended release formulation of Niacin, with BMSC not only significantly decreased adverse effects like BBB leakage, vascular damage, Angiogenin, matrix metalloproteinase 9 (MMP9) and ED1 expression, but also improved white matter remodeling and synaptic plasticity that are beneficial to recovery (Yan et al. 2013; Ye et al. 2013). Following 2 h MCAo, the animals were treated with BMSCs at 24 h and daily for 14 days orally with Niaspan. Niaspan is effective in reducing neurological deficits post stroke by promoting axonal remodeling, angiogenesis, and arteriogenesis (Chen et al. 2007, 2009; Cui et al. 2010; Yan et al. 2012). Another pharmacological drug that has been used in combination with BMSC is Sodium Ferulate (Zhao et al. 2013). This combination therapy significantly decreased infarction size and promoted exogenous BMSCs differentiation into neural-and astrocytic-like cells, as well as enhanced repair capacity of brain parenchymal cells by promoting glucose metabolism and endogenous neurogenesis after stroke. Combination treatment of stroke using BMSCs with statins also enhances BMSC migration into the ischemic brain, amplifies arteriogenesis and angiogenesis, and improves functional outcome after stroke (Cui et al. 2009; Pirzad Jahromi et al. 2012) .
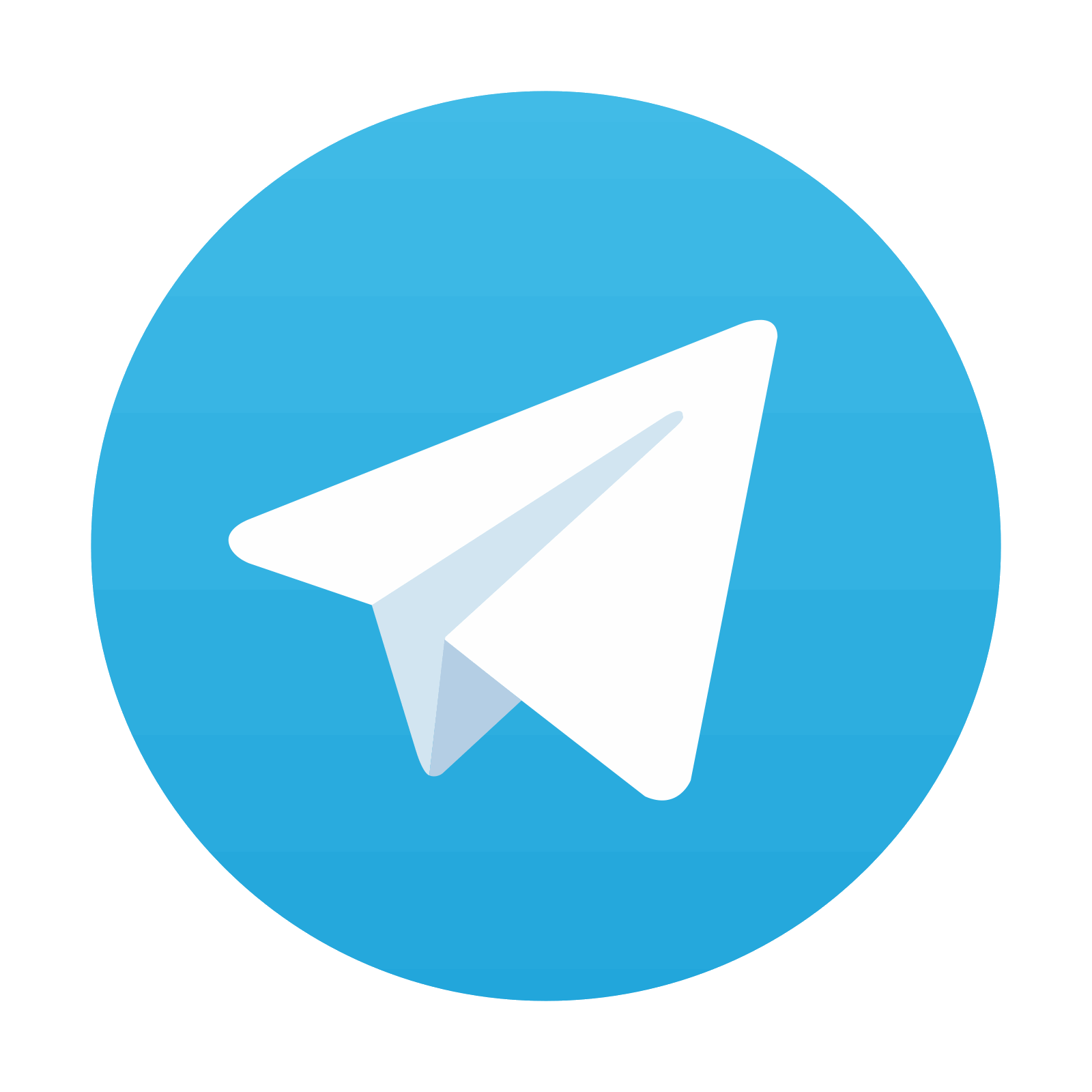
Stay updated, free articles. Join our Telegram channel
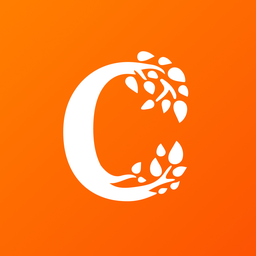
Full access? Get Clinical Tree
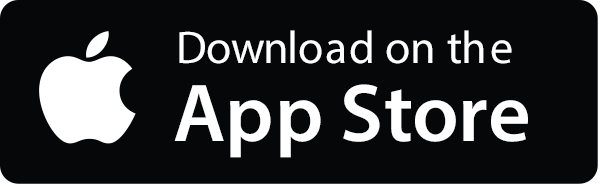
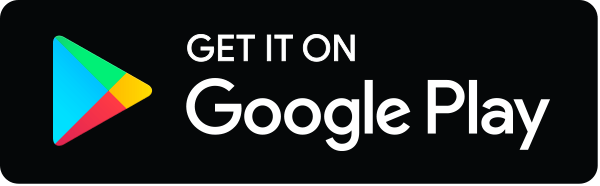