Transcranial magnetic stimulation (TMS) is a novel brain stimulation technique that has advanced the understanding of brain physiology, and has diagnostic value as well as therapeutic potential for several neuropsychiatric disorders. The stimulation involves restricted cortical and subcortical regions, and, when used in combination with a visually guided technique, results in improved accuracy to target specific areas, which may also influence the outcome desired. This article reviews the principles underlying the mechanism of action of TMS, and discusses its use to obtain functional maps of the motor and visual cortex, including technical considerations for accuracy and reproducibility of mapping procedures.
Localization of eloquent brain regions has evolved in the past few decades with the development of noninvasive techniques for cortical stimulation. Identification of eloquent brain areas such as the motor cortex is highly relevant in various fields ranging from preoperative planning of surgical procedures to studies of functional recovery following stroke, and administration of treatments such as repetitive transcranial magnetic stimulation (TMS) for the treatment of depression. Since its initial description in 1985 by Anthony Barker, TMS has evolved significantly. Initial studies involved blind placement of stimulating coils over the scalp surface. However, the advent of navigation systems using model reconstructions of the brain (mostly based on brain magnetic resonance imaging [MRI]) allowed for more precise positioning of coils during brain stimulation using TMS. Prior studies have shown that this approach reduces significantly the variability of brain regions stimulated, and has significant implications for the diagnostic and therapeutic use of TMS. TMS is considered a safe, noninvasive technique allowing also for assessment of various brain functions. This article reviews the principles underlying the mechanism of action of TMS, and discusses its use to obtain functional maps of the motor and visual cortex, including technical considerations for accuracy and reproducibility of mapping procedures.
Principles of TMS
In the early 1800s, Michael Faraday described the phenomenon of electromagnetic induction, in which an electric current induces a magnetic field, and a magnetic field in turn can induce electrical currents. It was not until 1985 that further use of magnetic stimulation was developed by Barker and colleagues, showing that magnetic pulses applied over the scalp elicit motor responses that can be used for transcranial motor cortex stimulation in a safe and painless fashion.
The magnetic stimulator consists of a component that generates, stores, and releases rapidly a large electrical current pulse through a copper coil, which in turn produces a pulsed magnetic field around the coil windings. The magnetic field then travels in the space underlying the coil, and, if it is impressed on electrically conductive tissue of sufficient volume, a secondary electrical current loop is generated within the tissue, much as in the secondary windings of a transformer. Such current loops are responsible for excitation of neural elements.
TMS stimulates different components of neural tissue, but a predominant descending motor path originating in the motor cortex that is excited by TMS is the corticospinal tract (CST). Controversy remains concerning the precise site of action of TMS and its ability to excite CST neurons directly. Some investigators have suggested that CST excitation is mediated by excitation of cortical interneurons. The latency of motor-evoked potentials (MEP) at all stimulus intensities produced by TMS have a longer latency than those seen using transcranial electrical stimulation (TCES). Day and colleagues, Rothwell and others have reasoned that, because TMS-induced current loops are parallel to the inner cranial surface, they would likewise parallel the surface of the cortical gyral caps. Thus, the current loops would be oriented along the axis of cortical interneurons, an orientation appropriate for cortical interneuron excitation. However, the induced current loops would be orthogonal to CST neurons, which are radially oriented within the cortical gyral caps. This current loop orientation is much less efficient for direct stimulation of CST cortical neurons.
However, other investigators have shown that direct TMS activation of CST neurons was possible with appropriate tilting of the stimulating coil on the scalp. The change in coil orientation on the scalp presumably led to induced current loops that paralleled the orientation of CST neurons (or the neuronal axons) within the cortex. Jalinous showed that large-diameter stimulating coils produce electric fields of greater intensity as a function of cortical depth than smaller coils. A scalp vertex orientation of a large round coil should, theoretically, cause direct excitation of CST cells located within the anterior bank of the central sulcus. At this location, these cells are oriented in a radial direction to both the cortical surface and induced current loops. Amassian and colleagues reasoned that magnetically induced current flowing within the axons tends to exit at the axonal bend, leading to a situation favorable for axonal excitation. Their results were consistent with CST activation deep within the cortex at the junction of the cortex and underlying white matter. They hypothesized that impulse initiation occurred near the axon hillock region or the CST basal dentrites. Rudiak and colleagues, using an analogous approach, found a similar depth for TMS excitation of geniculocalcarine fibers near the primary visual cortex or possibly within the secondary visual cortex.
Motor cortex mapping
TMS applied to restricted areas of the scalp overlying the motor cortex elicits MEPs in contralateral muscles, which may be used to map the location and extent of motor cortical representations for several muscles. Such maps are useful to assess normal motor cortical function as well as brain plasticity as a result of skill acquisition or pathologic lesions. They may also allow evaluation of brain response to injury (eg, as in stroke recovery), providing a tool to evaluate interventions that enhance recovery, assessment of patients with lesions near eloquent cortex before surgical interventions (eg, presurgical planning for resection of brain tumors near the motor cortex), and evaluation of cortical excitability in diseases affecting the nervous system.
Precision in targeting specific brain regions using TMS is of utmost importance. The functional patterns of the cortical surface are known to vary among individuals. Therefore, a cortical mapping technique should relate cortical surface anatomy and function.
Precision of TMS for Brain Mapping
The focality of a coil varies depending on its shape. Cohen and colleagues, using an experimental model, calculated the largest current densities occur under a stimulating coil, showing that a double circular coil produces the greatest focality with respect to magnetically induced stimulating currents. The figure-of-eight coil shows a peak induced electric field under the juxtaposition of the small circular components. The electric field is minimal at the center of each circular coil and has smaller peaks compared with the central contact of the 2 circular coils under the outer wing windings. The magnetic field was largest 3 cm from the center of the butterfly coil with a polarity reversal beyond the borders. Epstein and colleagues used 2 different-sized figure-of-eight coils to study the location of TMS-induced direct excitation of CST neurons in humans. A small magnetic coil produces a more intense magnetic field close to the coil surface than is the case with a larger coil. However, the magnetic field attenuates faster with distance from the smaller coil. Because CST neurons have the same threshold for activation, the locus for CST action potential initiation is the same for both types of coil. However, the magnetic coil current intensities are greater for threshold impulse initiation when using the smaller coil. Epstein and colleagues determined the depth within the cortex where CST impulse initiation occurred by knowing the degree of the induced electric field attenuation with distance and the threshold for producing a thumb twitch. Their results were consistent with CST activation deep within the cortex located at the junction of the cortex and underlying white matter. They hypothesized that impulse initiation occurred near the axon hillock region or the CST basal dendrites.
Ueno and Matsuda studied the resolution of TMS using a figure-of-eight coil. Single monophasic induced cortical current pulses were used. By optimizing the stimulation technique for focal excitation of the motor cortex distal upper limb foci, he was able to show a resolution of 0.5 cm for discrete TMS muscle activation.
Traditionally, TMS has been delivered using skull/scalp landmarks, in a blind fashion, holding the TMS coil in place while stimulation is delivered. Some have used head frames to immobilize the person’s head ; others mark the coil contour on a swimming cap worn by the individual being stimulated ( Fig. 1 ). However, displacement of the coil may occur, resulting in stimulation of different brain regions from those intended, and potentially affecting the results of the intervention.

We recently described a TMS mapping technique using stereotactic optic guidance. The purpose of this technique is to facilitate visualization of the cortical surface, and to guide placement of the TMS coil relative to the cortical surface of an individual. Thus, specific brain regions can be stimulated while controlling for coil movements relative to the person’s brain. The mapping procedure is described in the Appendix . In a previous study, we evaluated the precision attained using this optical tracking system using single-pulse TMS in normal volunteers. A blind technique, using markings of the figure-of-eight contour on a swimming cap worn by the volunteer to guide coil placement over the scalp, was compared with a guided technique, using the optical tracking system described. Three levels of TMS stimulation intensity were studied. The accuracy of coil repositioning at the optimal location for the first dorsal interosseus muscle was evaluated using a blind versus guided approach. Use of the guided technique resulted in smaller cortical regions stimulated ( Fig. 2 ). In addition, the distances between the cortical optimal locus and loci stimulated during subsequent stimulation trials were significantly smaller using the guided technique (<2 mm compared with 10–14 mm using a blinded technique). The differences of the three-dimensional (3D) angles achieved during the initial stimulation at the optimal location and subsequent trials attempting to reproduce it were also significantly smaller using the guided technique. These findings were more evident at lower stimulation intensities, as expected, because higher stimulation intensity is associated with current spread, and responses may be elicited by stimulating a location further from the intended target cortical locus. This study also showed that guided stimulation resulted in higher probability of eliciting MEPs, and that the MEPs obtained were of higher amplitudes and areas, although the coefficients of variation did not decrease significantly. This study also showed significant improvement in the precision to deliver TMS to target cortical regions, and illustrated some of the physiologic consequences.

Other investigators have also found improvement in precision for scalp placement of a coil by using other methods such as a 3D digitizer, or a chin rest and clamping the coil. However, the system developed by our group allows quantification of error in coil placement, real-time optical guidance for stimulation, and is frameless, allowing for greater patient comfort. Optical guidance to assist TMS studies improves the precision for coil placement.
Replication of TMS
A second important consideration when obtaining motor cortical maps is the reproducibility of the maps over time, which is relevant for instances such as the use of TMS in presurgical planning and the study of brain plasticity. Prior studies have investigated this aspect. Some limitations of these studies include the use of blind techniques, limiting the mapping procedure to the pericentral cortex in a single session, and limited control of coil placement.
We evaluated this question in a recent study, using the optical tracking system described earlier. In 2 mapping sessions performed 1 month apart, 8 healthy volunteers were stimulated using single-pulse TMS to obtain the functional representation for the first dorsal interosseus (FDI) muscle. After performing a coregistration procedure and calibration of the coil, a grid with intersections every 1 cm was displayed and projected onto the cortical surface ( Fig. 3 ). Although the grid was flat, the resulting distance between stimulated cortical loci was less than 1 cm. The coil was placed at each grid intersection visually guided by the tracking system, TMS was delivered, and MEPs recorded for offline analysis until no additional responses were obtained. We used a stimulation intensity set at 85% of stimulator output for mapping, based on prior experience that showed that this intensity is greater than the resting motor threshold for reliably eliciting responses from distal upper limb muscles. In addition, less intracortical current spread is expected than if using 100%. Three TMS stimuli were applied at each intersection point and the coil position was recorded. The MEPs were averaged at each intersection and a 4-point interpolated color cortical surface and scalp topographic map was constructed using the MEP amplitudes as a function of their cortical surface location ( Fig. 4 ).
The second mapping session followed the same process (ie, coregistration of the subject’s head and calibration of the coil, followed by retrieval of the initial map). The display in the monitor showed the coil orientation achieved during the initial map every time TMS stimuli were delivered. The coil position was shown represented by vectors ( Fig. 5 ). Next, the coil was placed on the scalp and, as soon as it was detected by the optical tracking cameras, vectors representing the coil location were displayed. The attempt was then made to match the coil orientation achieved during the first mapping session (by matching the vectors in the display). Once matching of coil placement occurred, TMS was delivered and the MEPs obtained recorded as well as coil position information. This procedure was repeated until all the loci stimulated in the first session were stimulated.
Using this system, accurate coil repositioning could be achieved. Comparison of the coordinates of cortical loci stimulated in the 2 mapping sessions showed excellent prediction of loci stimulated during a second mapping session based on the coordinates achieved in the first session. The system achieved an error of less than 3 mm (distance between cortical loci stimulated in the 2 sessions) in about 95% of the stimulation trials in the study, with a 3D angle difference of less than 3 degrees in about 85% of the trials. Evaluation of the physiologic responses, by comparing the MEPs obtained in the 2 mapping sessions, revealed no significant differences between MEP latency, amplitude, and area between the 2 sessions. The system again increased the probability of obtaining MEPs. Map response concordance rate was 0.86, reflecting the probability of either eliciting or failing to elicit MEPs in cortical loci stimulated in the 2 mapping sessions. In addition, comparing the motor map parameters revealed no significant differences between map area, volume, and amplitude at the optimal location in the 2 mapping sessions ( Table 1 and Fig. 6 ). The resting motor threshold at the optimal location was not significantly different between mapping sessions. The distance of the amplitude-weighted center of gravity in the 2 maps was 2.6 (±1.3) mm (mean ± standard deviation) across subjects. Table 1 summarizes the map parameters obtained in the 2 mapping sessions.
Map 1 | Map 2 | |
---|---|---|
Area (cm 2 ) a,b | 14.63 ± 8.52 | 15.75 ± 8.88 |
Volume a,c | 384.98 ± 391.06 | 381.23 ± 330.71 |
Maximal amplitude (mV) a,d | 2.23 ± 1.25 | 2.82 ± 1.59 |
Resting MT a,e | 70.2 ± 3.7 | 69 ± 3.38 |
Distance between amplitude-weighted CoG (mm) a,f | 2.6 ± 1.3 | |
Distance between optimal loci (mm) | 10 ± 5.5 |
a No statistically significant differences were found across sessions.
b Area refers to the number of excitable grid loci.
c Volume of the map refers to the sum of the relative MEP amplitudes (%M) across all excitable locations.
d Maximal amplitude refers to the highest peak-to-peak amplitude MEP elicited.
e The resting MT is expressed as percentage (%) of magnetic stimulator output.
f The x, y, and z coordinates for the CoG were recalculated by multiplying the coordinate at each position by its amplitude weight and summing overall positions stimulated.
Other important observations were made. First, some of the subjects showed several cortical loci where large MEPs were obtained, with amplitudes close to that obtained at the optimal location. This finding likely reflects the organization of the motor cortex in neuronal clusters that are closely arranged. This is a relevant consideration because using TMS in a blind fashion may target different brain areas that may elicit similar responses (at least in the motor cortex). Second, there were prominent interindividual differences in the area of motor maps between subjects. This finding is consistent with prior studies, and is an important consideration further supporting the use of a guided mapping technique.
Guided TMS using an optical tracking system resulted in reliable reproduction of coil placement and motor maps that were stable in the short term in a group of normal volunteers. Other studies support these findings. Mortifee and colleagues reported that motor maps were replicable for a range of 21 to 132 days on 6 normal volunteers. Thus, maps with greater variability might represent abnormalities or plastic changes in the brain as a result of adaptation to underlying disorders or physiologic adaptation, for instance during skill learning.
Future applications of the technique described for mapping the motor cortex include use in studies using TMS applied to brain regions other than the motor cortex. In addition, correlation with other mapping methods will provide further clarification of the best method to obtain function cortical maps.
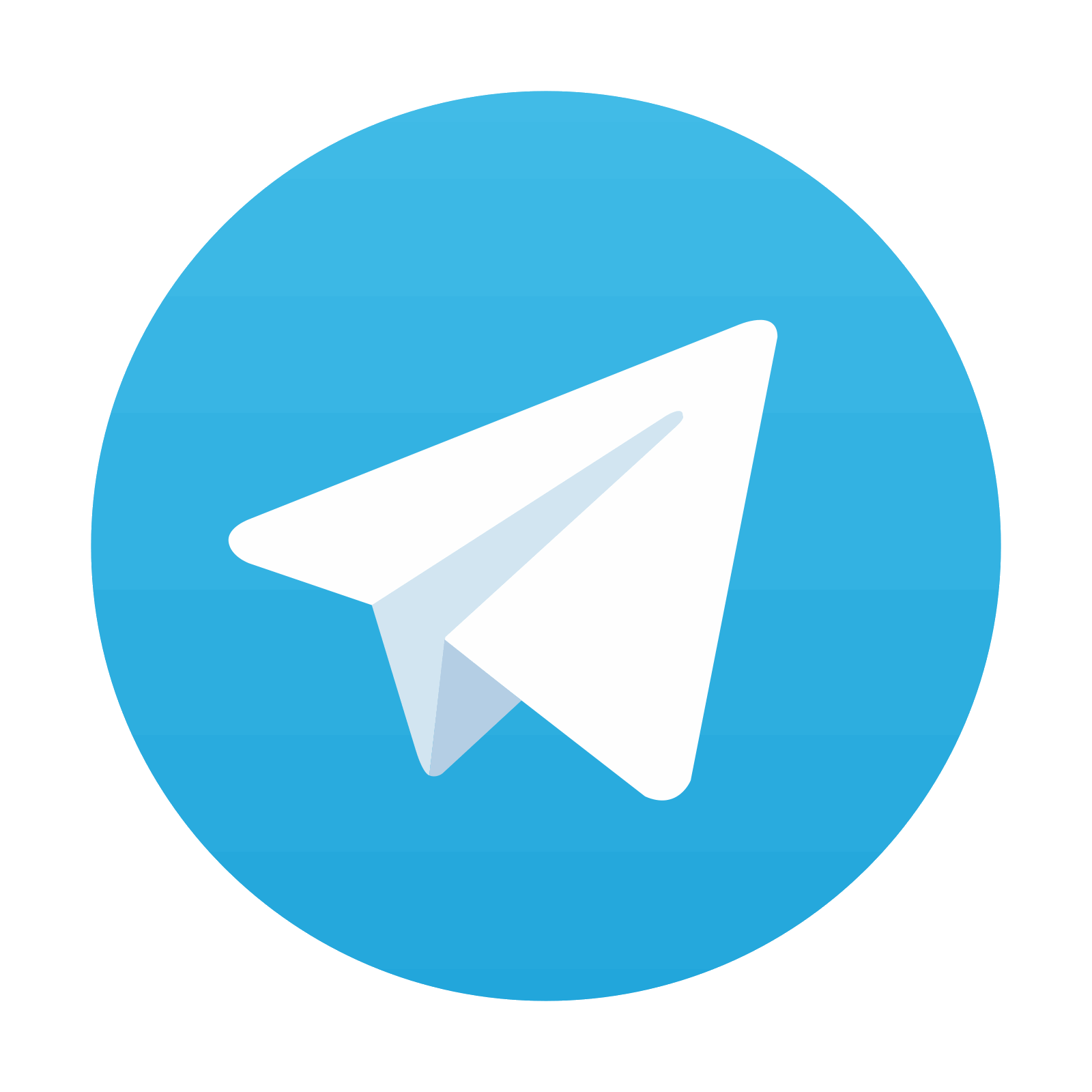
Stay updated, free articles. Join our Telegram channel
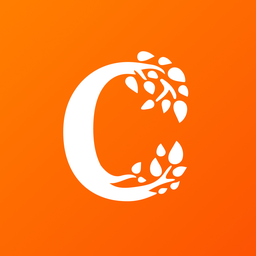
Full access? Get Clinical Tree
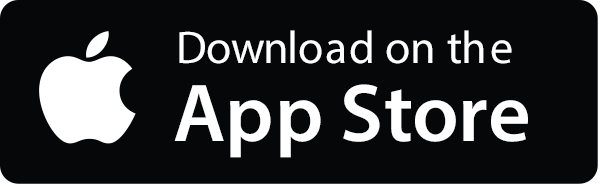
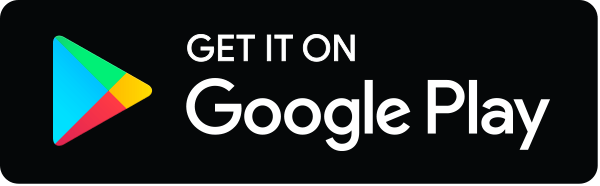