Fig. 2.1
A schematic diagram of two neural routes that mediate peripheral organ–brain interactions: (A) The afferent vagus–nucleus tract solitarii (NTS) axis. Information on the visceral organs is transmitted to the parabrachial nucleus (PBN) via the NTS, which is then transferred to the thalamus (the projection site is the medial portion of the ventroposterior thalamic complex), and finally to the cerebral cortex (mainly a posterior part of the insular cortex). (B) The sympathetic–spinal axis. Sympathetic afferents, in response to nociception related to mechanical, chemical, or thermal stimulation, transmit information to particular spinal neurons in the dorsal horn of the gray matter, and these neurons directly project to thalamic neurons in another part to which the vagus–NTS axis projects (the lateral part of the “shell” of the ventroposterior thalamic complex). Spinal neurons also project to the NTS and PBN by means of axon collaterals. The posterior insular cortex is the main projecting site of such thalamic neurons
The common hepatic branch of the vagus diverges from the left subdiaphragmatic vagus trunk passing along the esophagus (Berthoud and Neuhuber 2000; Puizillout 2005), immediately caudal to the diaphragm. The common hepatic branch is made up of about 3000 fibers, and afferent fibers occupy 2200 of them. There are only 200 efferent fibers, the remaining 600 are non-vagal adventitial fibers such as sympathetic postganglion or dorsal root afferent fibers (Prechtl and Powley 1990). The common hepatic branch of fibers further diverge and they project to the liver, bile duct, portal vein, and paraganglia (Berthoud et al. 1992); the remaining fibers form a gastro-duodenum branch (Berthoud and Neuhuber 2000; Puizillout 2005; Berthoud et al. 1992). The heterogeneity of the fibers in the common hepatic branch is important as described below. At least in the rat liver, vagal afferent axons terminate at the connective tissue surrounding intrahepatic triads, and their primary target is likely not to be the liver parenchyma (Berthoud and Neuhuber 2000; Berthoud et al. 1992). However, it seems that there is a species difference with respect to the target site (Metz and Forssmann 1980).
Generally, it is thought that vagal afferent fibers project to neurons in the nucleus tractus solitarii (NTS), the projection site in the CNS (Paton 1999). From the NTS, there is a direct connection to several brain stem nuclei; projection to the parabrachial nucleus (PBN) is particularly important as a relay pathway to the thalamus (Berthoud and Neuhuber 2000). It is thought that glutamate is a principal neurotransmitter released from vagal afferent terminals in the modulation of respiration, cardiovascular reflexes, gastrointestinal dynamics, and information about types, quantity, and location of nutrients such as lipid, carbohydrate, and protein (Hornby 2001; Travagli et al. 2006; Zheng et al. 2005; Ritter 2011; Julio-Pieper et al. 2011; Kline 2008). The mechanism in which only one kind of transmitter, glutamate, classifies information with different modalities is not yet understood, but one possible explanation is that afferent fibers originated in one internal organ project to particular NTS neurons with similar function and projection. However, a viscerotopic distribution of postsynaptic NTS neurons with different functions is not known (Paton 1999). For example, physiologically characterized neurons responding to distinct afferent modalities are very close neighbors and form neither laminar structure nor cluster (Paton 1999). On the other hand, it is known that viscerotopic distribution can be found in the same modality: for example, gustatory afferents project in the rostral part of NTS but gastrointestinal afferents localize more caudally (Altschuler et al. 1989). In addition to glutamate, neuromodulators that uniquely regulate vagal afferent from particular internal organs have been found. These are substance P (Sekizawa et al. 2003; de Lartigue 2014), nitric oxide and GABA in the case of regulation of cardiovascular functions, tachykinin and neurokinin A in the case of regulation of respiration, and cocaine and amphetamine regulated transcript (CART) and melanin concentrating hormone (MCH) in the control of food intake (de Lartigue and Raybould 2011; de Lartigue 2014).
Similarly to vagus afferent fibers innervating other organs, the CNS axon of the vagus nerve innervating the liver is known to project to the NTS (Rogers and Hermann 1983) in the rat. Detailed projecting sites are the left subnucleus gelatinosus, the medial division of the left solitary nucleus, and the left lateral edge of the area postrema (Rogers and Hermann 1983). Another study, however, could not find any axonal labeling in the NTS after injection of a transganglionic tracer, cholera toxin β-subunit–horseradish peroxidase, into the hepatic nerve, ileo-colic vein, portal vein wall, hepatic hilus, and hepatic parenchyma (Shin and Loewy 2009). An early electrophysiological study in the rat suggests that stimulation of the hepatic branch elicits responses in the left medulla with long latencies (Adachi 1981, 1984). The reason for long latencies is thought to be hepatic vagal branches comprising large numbers of non-medullated fibers (Adachi 1984). There are no data on the neurotransmitter of the vagal afferent innervating the liver.
Vagotomy has frequently been used to assess whether particular biological changes are mediated by the vagus nerve. Vagotomy of the common hepatic branch, in particular, has been extensively used, but care needs to be taken when interpreting the results from this method, largely due to heterogeneity of this branch (as mentioned earlier). In addition, the existence of another vagus branch that innervates the liver through the dorsal (right) subdiaphragmatic vagus trunk has been reported (Jancso et al. 1977; Niijima 1983; Berthoud et al. 1992; Phillips et al. 1997), which makes the interpretation of hepatic branch vagotomy more difficult.
It is known that capsaicin destroys unmyelinated afferents of both dorsal root and nodose ganglions (Jancso et al. 1977; Nagy et al. 1981; Chung et al. 1990; Holzer 1991; Carobi 1996) via the VR-1 vanilloid receptor (Michael and Priestley 1999). In the case of the vagus nerve innervating the liver in the rat, neonatal capsaicin treatment is known to completely destroy the smallest fibers (up to 180 μm2) innervating the left and median lobes and the right and caudate lobes of the liver (Carobi and Magni 1985). In addition, similar treatment reduced the number of neurons (up to 300 μm2) (Carobi and Magni 1985). Although there is no guarantee that all vagus afferents are capsaicin sensitive (Berthoud et al. 1997), capsaicin treatment may be an effective means of making vagus afferents innervating the liver inactive.
2.2.2 Chemical Substances That Change the Activity of the Vagal Hepatic Branch
As a result of neurons transmitting their activity by generating electrical spikes, the chemical substance that facilitates or suppresses vagal nerve spike activity is a candidate interoception mediator. There are several endogenous chemical substances that facilitate or suppress the spontaneous firing of this nerve, such as those listed in the following subsections (see also Table 2.1). It is important to keep in mind that the studies described in the following subsections are conducted using the common hepatic branch of the vagus nerve. This nerve bundle is composed not only of nerves that innervate the hepatoportal region but also nerves that innervate outside this region, mainly the gastrointestinal region (Horn and Friedman 2004), as mentioned earlier. Therefore, the possibility that activity may originate in fibers other than those from hepatoportal regions cannot be completely excluded.
Table 2.1
Endogenous compounds that modulate electrical activity of the common hepatic branch of the vagus nerve in rodents
Compound | Action | Route | Reference |
---|---|---|---|
Alanine, arginine, histidine, | + | PV (10 mM) | Niijima and Meguid (1995) |
leucine, lysine, serine, | |||
tryptophan, valine | |||
Cysteine, glycine, | _ | PV (10 mM) | Niijima and Meguid (1995) |
isoleucine, methionine, | |||
proline, threonine | |||
Linoleic acid | + | JI (1 ml/45 s) | Randich et al. (2001) |
+ | PV (1.5 mg) | Randich et al. (2001) | |
D-glucose | _ | PV (<100 mg/kg) | Niijima (1982) |
_ | DI (5 %, 5 ml) | Niijima (1982) | |
[glucose] in PV↑ | |||
Insulin | + | IV | Niijima (1984) |
Glucagon | _ | IV | Niijima (1984) |
GLP-1 | + | PV (0.2 pmol) | Nishikawa et al. (1996) |
CCK-8 | + | IV (2 μg/kg) | Cox and Randich (1997) |
IL-1β | + | PV (100 pg) | Niijima (1996) |
Somatostatin | + | PV (3.05 pmol) | Nakabayashi (1997) |
Amino Acids
When injected into the hepatic portal vein, some amino acids cause the firing rate in the hepatic branch of the vagus nerve to increase, although it takes 60 min or more to reach the maximal response (Niijima and Meguid 1995). Amino acids with such enhancing activity are alanine, arginine, histidine, leucine, lysine, serine, tryptophan, and valine. On the other hand, cysteine, glycine, isoleucine, methionine, proline, and threonine suppress the activity of vagus nerves (Niijima and Meguid 1995). The mechanism by which these amino acids elicit their activity is unclear but amino acid sensors on the vagus nerve are supposed to exist (Niijima and Meguid 1995).
Fatty Acids
It is known that linoleic acid injected into the hepatic portal vein increases hepatic vagus activity (Randich et al. 2001). Linoleic acid is an essential fatty acid and omega-6 polyunsaturated fatty acid. The action of linoleic acid on the vagus nerve is much slower than that of CCK-8 (2 μg/kg injected into the hepatic portal vein), in that it takes more than 30 min to reach the maximum response in the case of linoleic acid but less than 10 s in the case of CCK-8.
D-Glucose
The rate of afferent discharges recorded from the hepatic branch of the vagus nerve in the guinea pig is dose-dependently decreased by injection of D-glucose in the hepatic portal vein. Similar injection of other sugars, D-mannose, D-fructose, D-galactose, L-glucose, D-xylose, or D-arabinose, failed to induce changes in the discharge rate (Niijima 1982). Therefore, it is known that there is an inverse relationship between the activity of glucose-sensitive hepatic vagal afferents and glucose concentrations.
Insulin and Glucagon
Consistent with the results of glucose (mentioned above), the rate of discharge of hepatic vagal afferents in the rat is facilitated following administration of insulin and inhibited after application of glucagon (Niijima 1984). The so-called “hepatoportal glucose sensor,” the structure of which is not completely defined, is thought to be located upstream of the hepatic hilus and negative for the involvement of hepatocytes (Niijima 1984; Adachi et al. 1984). It is reported, on the other hand, that hypoglycemic signals in the portal vein are mediated by sympathetic afferents, because celiac-superior mesenteric ganglionectomy (but not hepatic branch vagotomy) abolishes the consequence of hypoglycemic signals (Fujita and Donovan 2005).
Glucagon-Like Peptide-1 (GLP-1)
A truncated form of GLP-1 administered into the hepatic portal vein (0.2 pmol) facilitates hepatic vagal afferent activity. Since the administration of 4.0 pmol elicited a similar facilitation, it seems that 0.2 pmol GLP-1 is sufficient to being about maximal response (Nishikawa et al. 1996). On the other hand, a similarly administered glucose-dependent insulinotropic polypeptide does not facilitate hepatic vagal afferent activity (Nishikawa et al. 1996).
Cholecystokinin-8 (CCK-8)
CCK-8 (2 μg/kg) also increases hepatic vagal afferent activity (15 of the 28 fibers tested, 54 %) as a result of intravenous administration via the CCKA receptor (Cox and Randich 1997). The response to CCK-8 injected in this way also occurs very quickly (within 10 s).
Interleukin-1β (IL-1β)
Injection of IL-1β (an inflammatory factor) into the hepatic portal vein facilitates firing of the hepatic vagus nerve in the rat. One shot of 100 pg IL-1β elicited gradual increase in spike frequency over 60 min (Ek et al. 1998). Intravenous injection of IL-1β also activates vagal afferents. It can be demonstrated that somata and/or fibers of sensory neurons of the vagus nerve express receptors to IL-1 and prostaglandin E2, and that circulating IL-1 stimulates vagal sensory activity via both prostaglandin-dependent and prostaglandin-independent mechanisms (Ek et al. 1998).
Somatostatin (SS)
SS (3.05 pmol, a physiological dose) injected into the rat portal vein increases the spike discharge rate in hepatic vagal afferents (Nakabayashi 1997).
2.2.3 Interoception in Metabolism
A likely indicator of brain–liver interaction is when metabolism in the liver is altered (Fig. 2.2A). For example, liver-specific disruption of peroxisome proliferator-activated receptor γ (PPARγ) in leptin-deficient obese mice prevents hepatic steatosis, but increases peripheral adiposity to aggravate diabetic phenotypes in the mouse (Matsusue et al. 2005). In contrast, tissue-specific overexpression of PPARγ in the liver induces severe hepatic steatosis, but peripheral adiposity is rather reduced as a result of enhanced lipolysis (Uno et al. 2006). A putative mechanism underlying these homeostatic changes between the liver and adipose tissues is participation of the autonomic nervous system. Namely, information is transmitted from the liver to the brain via neuronal and humoral factors, and then the brain controls adipose tissues using efferent sympathetic nerves. Indeed, the increase of lipolysis in brown adipose tissue (BAT) by overexpression of PPARγ in the liver is abolished by hepatic branch vagotomy (Uno et al. 2006). Furthermore, high-fat feeding induced hepatic glucokinase upregulation, and hepatic glucokinase gene overexpression dose-dependently decreased adaptive thermogenesis by downregulating thermogenesis-related genes in the BAT (Tsukita et al. 2012). This inter-tissue (liver-to-BAT) system is mediated by the afferent vagus from the liver to the brain and sympathetic efferents from the brain to the BAT (Tsukita et al. 2012). The liver–brain–adipose neural axis is found to have an important role in switching the fuel source from glycogen to triglycerides under prolonged fasting conditions (Izumida et al. 2013).
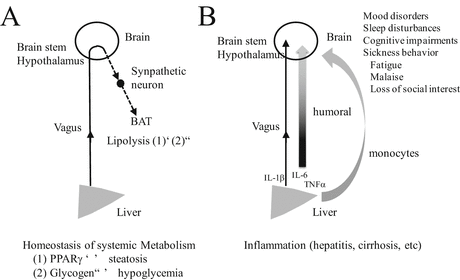
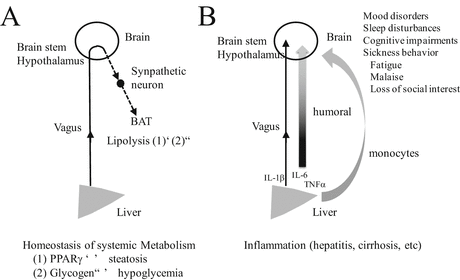
Fig. 2.2
Two potential pathways for liver–brain communication: (A) Homeostatic control of systemic metabolism. Overexpression of PPARγ in the liver induces steatosis of the liver, which facilitates lipolysis in brown adipose tissue (BAT) (1). On the contrary, decrease in liver glycogen reduces lipolysis such that lipids accumulate in BAT (2). These two homeostatic changes are mediated by vagus afferent fibers from the liver to the brain and sympathetic neurons from the brain to the BAT. (B) Effects of liver inflammation (in hepatitis or cirrhosis) on brain function. The vagus nerve innervating the liver can be activated by proinflammatory cytokines such as IL-1β, which may follow a neuronal route to influence the brain. Moreover, inflammation of the liver induces the release of cytokines IL-1β, IL-6, and TNFα into body fluid. These cytokines react with receptors on endothelial cells in the brain. Furthermore, an immune cell (a monocyte) transmigrates into the brain in response to initial activation of brain-resident microglia to produce a potent monocyte chemoattractant (MCP-1). Such liver–brain communications can induce changes in neural network activity in the brain. Changes in behavior that are induced result in mood disorders, sleep disturbance, cognitive impairment, and fatigue
2.2.4 Interoception in Inflammation
Another likely indicator of brain–liver interaction is the appearance of chronic liver diseases such as hepatitis and cirrhosis (Fig. 2.2B). Chronic inflammation of the liver is a disorder that is frequently associated with sickness behavior (fatigue, malaise, loss of appetite, loss of social interest, and so on) and alteration in mood state (D’Mello et al. 2009; Nguyen et al. 2007; Swain 2006; Swain and Maric 1995; Capuron and Miller 2011; Isik et al. 2007; Jones et al. 2006). In these disorders, significant pathological CNS tissue damage is basically absent (Forton et al. 2002, 2008; Pollak et al. 2003). A mechanism that explains CNS symptoms in periphery-originated diseases satisfactorily is that incorrect information about the state of peripheral organs is transmitted to the brain. It is thought that both neuronal and humoral factors are responsible for this and that vagus nerves are main players in neuronal factors (Goehler et al. 2000). In fact, the common hepatic branch of the vagus nerve can respond to proinflammatory cytokine IL-1β (as mentioned above) (Niijima 1996; Ek et al. 1998). The vagus nerve can be shown to express cytokine receptors such as the IL-1 receptor (Ek et al. 1998). In addition, endotoxin lipopolysaccharide (LPS), a gram-negative bacterial cell wall component, is a potent inducer of TNFα, IL-1β, and IL-6 (Dantzer et al. 2008; Capuron and Miller 2011; Lebbar et al. 1986; Vallieres and Rivest 1997; Laye et al. 2000). It is known that plasma endotoxin is elevated in patients with chronic liver disease (Yamamoto et al. 1994; Swain 2006). Therefore, cytokines released peripherally in response to bacterial endotoxin are thought to be important in communication between the brain and the liver through the vagus nerve. Consistently, in LPS-induced peritoneal inflammation, the NTS to which vagus afferents are projected, and subsequent regions to which NTS neurons project abundantly – the PBN and the hypothalamic paraventricular nucleus – are positive when assessed with expression of immediate early gene c-fos (Wan et al. 1993; Goehler et al. 2000). The intraperitoneal injection of IL-1β not only induces c-fos expression in the brain but also alters social exploratory behavior in the rat (Bluthe et al. 1996). It is known that changes in both c-fos expression and behavior can be reduced by subdiaphragmatic vagotomy (Bluthe et al. 1996).
Brain regions activated by inflammation were elucidated by getting healthy male volunteers (some of whom received typhoid vaccination or saline) to conduct a Stroop task during functional magnetic resonance imaging. Typhoid but not saline injection produced a robust inflammatory response and a significant increase in fatigue, confusion, and impaired concentration in participants. Performance of the Stroop task under inflammation-activated brain conditions involved the participation of the brainstem, thalamus, amygdala, cingulate, and bilateral mid and anterior insula in afferent interoception. Activity changes in these regions can be confirmed to be a result of inflammation (Harrison et al. 2009).
Dantzer et al. (2008) is an excellent review on the relation between inflammation and sickness behavior. The authors show that decompensation of sickness behavior could be a risk factor for mood disorders.
2.3 The Insular Cortex as the Destination of Interoception
A large part of the information collected by interoception generates the reflex to maintain the body’s homeostasis. The hypothalamus controls a vast array of these physiological processes including sleep/wake cycles, sexual behavior and reproduction, and metabolic control such as thermoregulation, energy intake/expenditure, glucose metabolism, lipid metabolism, and food and water intake. In many cases, sympathetic neurons play a central role in these reflex reactions. There are excellent reviews on the role that sympathetic neurons and the hypothalamus play in homeostatic control (Saper 2002; Kalsbeek et al. 2014). To avoid duplication, I would like to introduce here another brain structure, the insular cortex, as a major ascending destination of interoception.
The insular cortex is part of the cerebral cortex and is located deep in the lateral sulcus in humans. In rodents, it surrounds the rhinal fissure and is between the primary/secondary somatosensory and piriform cortices in the most anterior–posterior levels (Paxinos and Franklin 2001). The insular cortex is normally divided into three subdivisions (Cechetto and Saper 1987): the agranular insular cortex (AI), which surrounds the rhinal fissure; the granular insular cortex (GI), which is located just ventral to the secondary somatosensory cortex; and the dysgranular insular cortex (DI), which is between the AI and GI. Each subdivision is thought to involve sensory information, in particular. For example, the AI is thought to be involved in nociceptive and autonomic information processing (Cechetto and Saper 1987; Jasmin et al. 2003; Burkey et al. 1996, 1999); the GI plays a crucial role in modulating visceral function (Yamamoto et al. 1981, 1984); and the DI participates in gustatory processing (Cechetto and Saper 1987). It is known that ascending visceral afferents, which project from ventroposterolateral (VPL) and ventroposteromedial (VPM) parvicellular (pc) nuclei of the thalamus and from the PBN, are localized in the GI and DI (Allen et al. 1991). On the other hand, integrated limbic afferents from the infralimbic cortex and the mediodorsal nucleus of the thalamus project to the AI (Allen et al. 1991). The convergence of visceral and limbic information may be used by the insular cortex to integrate autonomic response with ongoing behavior and emotion.
2.4 Vagus Nerve Stimulation
Stimulation of the vagus nerve has been clinically utilized in the treatment of epilepsy and depression (Beekwilder and Beems 2010; Bonaz et al. 2013). The first attempt at vagus stimulation using an implanted electrode in humans was reported in 1988 for the treatment of drug-resistant epilepsy (Penry and Dean 1990). In 1997, vagus nerve stimulation was approved by the Food and Drug Administration (FDA) in the United States as an adjunctive treatment for drug-resistant seizures, and then in 2005 for depression. It can be demonstrated that the activation of vagus afferents suppresses seizure in rats (Krahl et al. 1998, 2001). The latter therapy is based on mood improvement in patients with epilepsy receiving vagus nerve stimulation (Groves and Brown 2005), but its effectiveness has not yet been confirmed by meta-analysis because of insufficient data (Martin and Martin-Sanchez 2012). Vagus nerve stimulation is effected by wrapping an electrode around the left vagus nerve in the neck (Reid 1990).
The precise mechanism underlying vagus nerve stimulation in epilepsy and depression is obscure, but it is postulated that vagus nerve stimulation modulates the activity of the following neuronal networks via the NTS (Bonaz et al. 2013): (1) activation of the thalamus and thalamocortical pathway; (2) suppression of the amygdala and hippocampus; and (3) increased norepinephrine and serotonin release in widespread cerebral regions. The locus coeruleus (LC), the main origin of noradrenergic projection, mediates at least some of the effects of vagus nerve stimulation in attenuating seizures because LC lesions suppress the antiepileptic effect of vagus nerve stimulation (Krahl et al. 1998). The LC is directly connected to the NTS. Activity mapping using c-fos in rats shows that positive signals are increased in the amygdala, cingulate, LC, and hypothalamus in response to antiepileptic stimulation of the vagus nerve (Naritoku et al. 1995). In humans, functional neuroimaging studies suggest that the thalamus, cerebellum, orbitofrontal cortex, limbic system, hypothalamus, and medulla exert long-term changes in their activity in response to vagus stimulation (Lomarev et al. 2002; Chae et al. 2003).
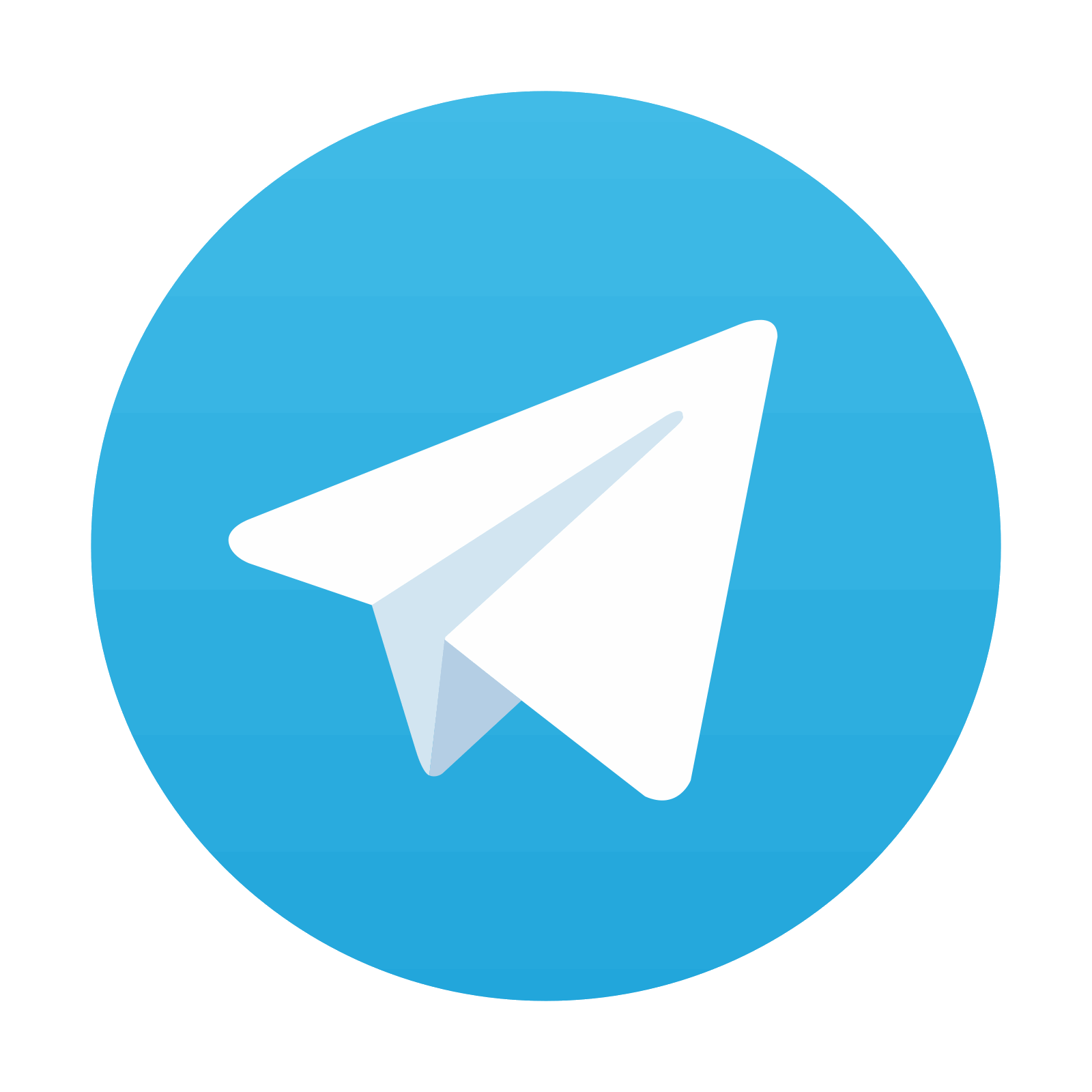
Stay updated, free articles. Join our Telegram channel
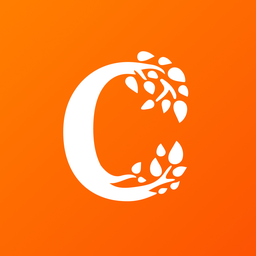
Full access? Get Clinical Tree
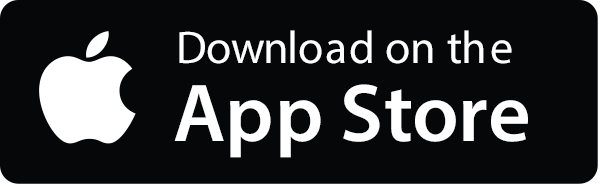
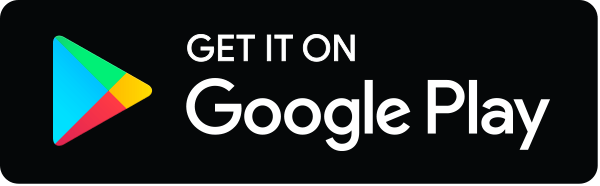