5 Marco Bacigaluppi, Erica Butti, Cecilia Laterza, Donatella De Feo, Luca Peruzzotti, Arianna Merlini, Melania Cusimano and Gianvito Martino Neuroimmunology Unit, Institute of Experimental Neurology (INSpe), Division of Neuroscience, San Raffaele Scientific Institute, Milan, Italy Inflammation and degeneration are typical pathological processes occurring in the central nervous system (CNS) of patients with chronic irreversible neurological conditions. They are only apparently separate processes because, as soon as the pathological process becomes chronic, they have the tendency to become strictly interrelated (Martino et al., 2002, 2011; Martino, 2004). Therefore, primary neurodegeneration triggers secondary inflammatory reactions, while primary inflammatory reactions lead to secondary neurodegeneration. In the last 50 years, it has become clear that the CNS displays intrinsic ‘constitutive’ tissue repair ability to prevent the irreversible tissue damage occurring as a consequence of chronic inflammatory and/or degenerative processes. Several molecular and cellular events sustaining innate brain repair mechanisms have been described. On one hand, humoral and cellular inflammatory components shift their function over time, from a tissue-damaging mode to one promoting tissue repair. Not only pro-inflammatory cytokines may, under certain circumstances, act as anti-inflammatory molecules, but also pro-inflammatory mononuclear cells may promote neuroprotection by secreting neurotrophic factors (Martino et al., 2002). On the other hand, the recruitment of alternative ‘non-damaged’ functioning neuronal pathways – occurring also via axonal sprouting and synaptogenesis – takes place as a consequence of brain damage. Whether or not this process is paralleled by central axon regeneration (i.e. an axon growing back along the distal stump of a crushed or transected nerve to re-innervate its normal target) is still a matter of intense debate (Tuszynski and Steward, 2012). Finally, endogenous neural stem and precursor cells – the self-renewing and multipotent cells of the CNS capable of driving neurogenesis and gliogenesis in adult life – may adapt targeted migration into damaged areas, to promote tissue repair and regeneration via cell replacement and/or the so-called bystander (paracrine) effect, which is due to the capacity of such cells to constitutively secrete neurotrophic and anti-inflammatory molecules (Martino et al., 2011). However, ‘protective’ mechanisms reactive to CNS damage are not strong enough to promote the full recovery of cyto-architecture in most of the chronic inflammatory and degenerative neurological disorders. This evidence supports the ensuing view that irreversible neurodegeneration might be a consequence of the failure of CNS intrinsic repair mechanisms. Thus, it is believed that fostering and/or resetting ‘spontaneous’ regenerative process may lead to more efficacious, and less toxic, therapies. Given that stem cells are an integral part of the mechanisms sustaining CNS tissue repair processes, strategies that mobilize neural progenitor cells (NPCs) or in vivo transplantation of NPCs might represent promising therapeutic approaches to foster intrinsic repair mechanisms and promote neuroprotection (Martino et al., 2010; Pera and Tam, 2010). In this chapter, we will focus our attention on the self-renewal and differentiation potential and on the recently discovered homeostatic constitutive functions of endogenous NPCs as prerequisites to develop NPC-based transplantation strategies aimed at promoting CNS repair. At the same time, we will also discuss the ability of transplanted NPCs to adapt behaviour and fate in response to the CNS microenvironment and how this behaviour culminates in protection of the CNS from pathogenic signals, the concept of ‘therapeutic plasticity’. In the adult rodent CNS, neurogenesis continues for the rodent’s entire life mainly in two regions, the subventricular zone (SVZ) of the lateral ventricles and the subgranular zone (SGZ) of the hippocampal dentate gyrus (Alvarez-Buylla and Lim, 2004; Zhao et al., 2008). Newly formed adult NPCs of the SGZ migrate short distances and differentiate into dentate granule cells (DGCs) within the dentate granule cell layer (Kallur et al., 2011). These newly formed DGCs migrate into the granule cell layer to become indistinguishable from pre-existing cells and are necessary for modulating and refining the existing neuronal circuits involved in hippocampus-dependent memory processing and behaviour (Imayoshi et al., 2008; Kitamura et al., 2009; Singer et al., 2011). In the rodent, however, SVZ NPCs migrate along the rostral migratory stream (RMS) to the olfactory bulb where they integrate within the granule and glomerular cell layer in order to maintain and reorganize the olfactory bulb system (Imayoshi et al., 2008). However, recent evidence challenges the sole and exclusive view that the neurogenic CNS areas mainly act as a source of newly formed neurons, able to replace neuronal cells in the hippocampus and in the olfactory bulb (Imayoshi et al., 2008). As a matter of fact, adult NPCs residing within germinal niches might exert other ‘non-neurogenic’ homeostatic regulatory functions alternative to cell replacement during either a healthy state or pathology. Apart from a recent report showing that neurogenesis in the SGZ exerts an antidepressant activity by regulating (via glucocorticoid release buffering) the hypothalamus–pituitary axis (Snyder et al., 2011), evidence collected so far suggests that SVZ represents the more ‘strategic’ area where NPCs might exert a homeostatic role in addition to neurogenesis. This is because, on one side, these cells are in communication with two different microenvironments, tightly apposing blood vessels and in contact with the cerebrospinal fluid (CSF) through apical processes (Sawamoto et al., 2006; Rolls et al., 2007; Mirzadeh et al., 2008; Tavazoie et al., 2008). On the other side, the SVZ is very close to crucial areas of the midbrain (e.g. the basal ganglia and striatal structures) containing GABAergic neurons capable of efficiently regulating and modulating interconnections between several cortical and sub-cortical brain areas (Koos and Tepper, 1999). As a matter of fact, SVZ NPCs protect striatal neurons from glutamatergic excitotoxicity, as seen in the early phase of ischaemic stroke and epilepsy, by releasing endogenous endocannabinoids, N-arachidonoylethanolamide (AEA) and 2-arachidonoylglycerol (2AG) capable of binding to their specific receptors (CB1 and CB2) (Butti et al., 2012). This NPC-mediated operational way of acting is tuned up during CNS-compartmentalized inflammatory insults. It is thus tempting to speculate that endogenous SVZ NPCs act as guardians of the brain, by sensing danger signals coming from the periphery and responding by down-modulating glutamatergic excitotoxic currents, whose deregulation might, in turn, be noxious for proper functioning of brain cells (see Figure 5.1). The above-mentioned strategic positioning of SVZ NPCs within the CNS supports this novel view while questioning the positioning as just representing a developmental relic. In addition, SVZ NPCs have been shown to be capable of exerting a physiological phagocytic activity that requires intracellular engulfment protein, ELMO1, to promote Rac activation downstream of phagocytic receptors (Lu et al., 2011). Finally, SVZ NPCs may mediate suppression of high-grade astrocytomas (HGA) by releasing endovanilloids that activate the transient receptor potential vanilloid subfamily member-1 (TRPV1) on HGA cells, triggering their death and thus prolonging overall survival time (Stock et al., 2012). Figure 5.1 Structural and functional characteristics of NPCs in the SVZ. Schematic representation of the functional homeostatic role exerted by NPCs in the SVZ and striatum. SVZ NPCs may sense danger signals, coming from either the CSF or the blood, via Toll-like receptors (TLRs). TLR4 is triggered by several types of endogenous and exogenous danger signals, including those released from neural cells stressed by glutamate-induced excitoxicity (e.g. heat shock protein 70, saturated fatty acids, high-mobility group box 1 proteins (HMGB1) and β-defensins). As a consequence of such triggering, SVZ adult neural precursor cells increase the production of the endogenous cannabinoid AEA (blue dots) which, in turn, binds to pre- and post-synaptic CB1 receptors. CB1 receptor binding decreases Ca2+ content within the presynaptic compartment, thus reducing glutamate release within the synaptic space. This regulatory mechanism reduces post-synaptic glutamatergic currents and glutamatergic-mediated excitotoxicity, thus representing an innate protective mechanism. While possibly explaining the role of SVZ NPCs in non-human primates and humans, where the presence of a fully formed RMS is not yet substantiated (Sanai et al., 2004, 2007), the homeostatic role of SVZ NPCs is also supported by recent evidence indicating a long-lasting neurogenic response reactive to injury. This has been observed in both patients (Marti-Fabregas et al., 2010) and animal models (Bengzon et al., 1997; Parent et al., 1997; Jin et al., 2001, 2010; Goings et al., 2004) affected by neurological diseases, but it is not yet confirmed whether such approaches replace damaged and/or dead cells. In stroke, about 80–90% of newly formed SVZ-derived cells die within a few weeks without integrating into the spared neuronal circuitries (Arvidsson et al., 2002), but they seem to protect against tissue injury through the secretion of neurotrophic factors in ischaemic CNS areas (Jin et al., 2010). In the toxin-induced cuprizone model in which demyelination of the corpus callosum is induced, less than 4% of newly formed SVZ-derived cells differentiate into myelinating oligodendrocytes (Menn et al., 2006). However, SVZ-derived newly formed NG2+ cells can still promote remyelination, by forming functional glutamatergic synapses with demyelinated axons (Etxeberria et al., 2010). In experimental epilepsy, SVZ-derived cells migrating towards the hippocampus terminally differentiate into glial but not neuronal cells (Parent et al., 2006). This is likely to exert a protective effect because in temporal lobe epilepsy newly born neurons aberrantly migrate and integrate in the dentate hilus, exacerbating the hippocampal epileptic activity (Hattiangady and Shetty, 2008). Although the replacement of damaged cells seems not to be the prevailing and sole mechanism of reactive neurogenesis occurring in response to tissue damage (at least in the SVZ), it is very likely that the specific characteristics of the pathological environment influence the behaviour of SVZ-derived newly formed cells. As such, inflammation occurring as a consequence of autoimmunity and/or traumatic and ischaemic injuries has been variably shown to alter NPC proliferation and differentiation characteristics in a non-cell-autonomous fashion. On the other hand, when inflammation fades away and neurodegeneration prevails, endogenous NPCs tend to differentiate into multiple neuronal lineages, depending on the situation, that are partly capable of integrating into damaged neuronal circuits (Kokaia et al., 2012). The increase in the numbers of microglia cells within the SVZ neurogenic area during inflammatory CNS conditions (Pluchino et al., 2008) supports this working hypothesis, as does recent evidence emerging from NPC transplantation studies, which will be discussed in detail in this chapter. Briefly, while remaining undifferentiated, transplanted SVZ-derived NPCs might promote CNS tissue healing via the secretion of immunomodulatory and neuroprotective molecules capable of reducing detrimental tissue responses, the so-called bystander effect (Ourednik et al., 2002; Pluchino et al., 2003; Martino and Pluchino, 2006a; Bacigaluppi et al., 2009a,b). As already anticipated, CNS pathology results in loss of either neural or glial cells and disrupts the cyto-architecture of the tissue that is defined during development, when neurons and glia precociously acquire specific positional identities (Hochstim et al., 2008; Sauka-Spengler and Bronner-Fraser, 2008) and their integrity is required for correct CNS functioning (Eroglu and Barres, 2010). Therefore, cell replacement strategies would represent an ideal therapeutic approach for CNS disorders, owing to the fact that it not only aims at substituting dead cells with newly differentiated ones but also promotes functional integration into neural circuits of newly formed cells (Ingber and Levin, 2007; Poss, 2010). With this in mind, several cell replacement–based therapeutic strategies have been established. The results, obtained so far, reveal that this approach does represent a rational and realistic therapeutic strategy for only a restricted category of neurological disorders, and the success of this strategy is very much dependent on the cell type to be substituted and on the CNS region damaged. Cell replacement is efficacious in disorders in which degeneration is caused by either intrinsic cellular defects of, or extrinsic factors that are no longer active in, a specific cell population residing within a discrete CNS area, but where the general architecture of the tissue is maintained. In animal models of Parkinson’s disease (PD), dopaminergic neuronal precursors – derived from different animal species (Dunnett et al., 1987; Wictorin et al., 1992; Isacson and Deacon, 1996; Starr et al., 1999) and cell sources (Dunnett et al., 1987; Shim et al., 2007; Wernig et al., 2008) – can survive, re-innervate the striatum and ameliorate clinical outcome, when grafted either in the substantia nigra or in the striatum (Gaillard and Jaber, 2011). In animal models of genetically induced dysmyelination and/or hypomyelination (i.e. shiverer mouse) (Ben-Hur et al., 2005; Duncan et al., 2011) or chemically induced demyelination (Blakemore and Franklin, 2008), intraparenchymal transplantation of many different myelinating cell types extensively remyelinates denuded axons (Windrem et al., 2004; Buchet et al., 2011; Sim et al., 2011). In contrast, cell replacement has been only partially satisfactory in a persistently unfavourable environment, where different cell sub-populations in different CNS areas are affected and where the tissue architecture is altered, such as in stroke, spinal cord injury (SCI), multiple sclerosis (MS) and amyotrophic lateral sclerosis (ALS) (Chen and Palmer, 2008). In stroke and SCI animal models, NPCs from multiple sources have been demonstrated to functionally integrate into the host neural circuits and differentiate into neurons (Kelly et al., 2004; Cummings et al., 2005; Buhnemann et al., 2006; Yan et al., 2007; Daadi et al., 2009; Braz et al., 2012). Likewise, in animal models of MS and SCI, both NPC and glial-restricted progenitors can remyelinate injured axons (Archer et al., 1997; Pluchino et al., 2003; Keirstead et al., 2005; Lee et al., 2009; Sharp et al., 2010; Yang et al., 2010). However, it still remains to be demonstrated if this cell replacement mechanism is the sole mechanism functionally accountable for the clinical amelioration (Dubois-Dalcq et al., 2005; Pluchino et al., 2005; Bacigaluppi et al., 2009b; Sahni and Kessler, 2010). Other mechanisms that will be discussed in this chapter (e.g. the bystander effect) may also contribute to the therapeutic effect observed upon NPC transplantation. Another issue is the degree of complexity of the tissue patterning that transplanted cells need to restore. PD and focal demyelination are diseases in which specific cell types are necessary to re-establish appropriate interactions at the cellular level; in PD dopaminergic circuits should be restored within the striatal region, while in demyelinating disorders a properly functioning saltatory conduction should be achieved via efficient remyelination. However, very few studies have been able to demonstrate that cell transplantation might lead to the generation of long-distance connections in diseases in which degeneration impairs multiple widespread afferent–efferent connections, such as ALS and other motor neuron disorders (Verstraete et al., 2011), Huntington’s disease (HD) (Wolf et al., 2008) or spinocerebellar ataxias (Di Giorgio et al., 2011; Yohn et al., 2008; Solodkin et al., 2011; Lepore et al., 2011). The last issue to be considered when designing therapies aimed at promoting cell replacement is the timing of cell transplantation. In primary neurodegeneration, transplantation should be performed at the beginning of the neurodegenerative process in order to limit the cascade of events leading to the degeneration of other cell populations. Data clearly show that transplanted NPCs acquire an appropriate terminally differentiated fate and integrate functionally in the host tissue (Goldman, 2005; Breysse et al., 2007; Lindvall and Kokaia, 2010), an event particularly evident when there is a partial preservation of the neuronal circuits’ cyto-architecture (Shihabuddin et al., 1996). On the other hand, while early transplantation is recommended in degenerative diseases, in primary inflammatory disorders (e.g. stroke, SCI and MS) transplantation aimed at cell replacement would be useful only during the post-acute phase of the disease (Karimi-Abdolrezaee et al., 2006; Bacigaluppi et al., 2009b) when the inflammatory phase is limited. It is now clear that the immune milieu can influence, either detrimentally or protectively, the fate of transplanted cells (Carpentier and Palmer, 2009; Deverman and Patterson, 2009; Ransohoff, 2009; Giannakopoulou et al., 2011; Muja et al., 2011; Kokaia et al., 2012). Despite the initial perception that transplantation of NPCs could serve to replace only damaged cells, recent experimental studies have shown that NPCs could exert a plethora of different neuroprotective effects, spanning from neurotrophic support to immunomodulation, when transplanted in chronic inflammatory neurological disorders. (See Figure 5.2.) Figure 5.2 Operational mechanism(s) of action of endogenous and transplanted NPCs. The extent of tissue regeneration driven by endogenous as well as transplanted multipotent NPCs is very much dependent on the different operational mechanism(s) of action that such cells adopt while interacting with CNS resident and blood-borne infiltrating mononuclear cells, homing within the microenvironment during both physiological and/or pathological circumstances. Both cell-autonomous and non-cell-autonomous mechanisms may affect the final fate and behaviour of endogenous and transplanted NPCs as well as their interactions with CNS resident and CNS-infiltrating cells. While cell-autonomous mechanisms driving terminal differentiation of exogenous versus transplanted NPCs tend to prevail in pathological conditions mainly characterized by neuronal degeneration and mild reactive inflammation (mainly driven by CNS resident microglia), non-cell-autonomous mechanisms mainly affect NPCs when acute or chronic non-resolving inflammation is ongoing. As a matter of fact, endogenous and transplanted NPCs sense the inflammatory environment and, within such an environment, might promote tissue homeostasis and repair by releasing at the site of tissue damage a milieu of constitutively expressed molecules (chemokines, cytokines, growth factors and stem cell regulators) capable of immunomodulation and trophic support, the so-called bystander effect. The bystander effect is made possible by the fact that inflammation inhibits NPC proliferation, thus maintaining NPCs in an undifferentiated state; this is the best situation possible for NPCs to release constitutively a wide set of neuroprotective molecules. However, cell-autonomous mechanisms, driving terminal differentiation during lineage commitment, limit the amount of molecules constitutively released by NPCs due to epigenetic restriction of transcriptional circuits. To what extent the interaction between the inflammatory microenvironment and transplanted NPCs results in protective versus detrimental effects is still far from being fully elucidated. Some of these ideas are discussed below. Firstly, NPCs show a certain degree of pathotropism towards inflammatory foci, which is due to the fact that such cells constitutively express an armamentarium of chemokines and chemokine receptors (e.g. CCR1, CCR5, CXCR3 and CXCR4), cell adhesion molecules (e.g. CD44) (Rampon et al., 2008) and integrins (e.g. VLA4) (Campos et al., 2004, 2006; Leone et al., 2008). Thus, transplanted NPCs are able to follow gradients of chemoattractants and reach multiple inflamed CNS regions when intraparenchymally and/or systemically injected (Ji et al., 2004; Pluchino et al., 2005). This is a very important issue in multifocal CNS inflammatory disorders where multiple intraparenchymal NPC injections are clinically impractical. Once migrated into inflamed CNS areas, transplanted NPCs survive within perivascular inflammatory foci (i.e. the atypical ectopic niche) where they interact with many other cell types such as CNS-infiltrating blood-borne inflammatory cells, endothelial cells and CNS resident astrocytes and microglia. Within these ectopic niches, inflammatory molecules (e.g. interferon gamma (IFNγ) and tumour necrosis factor alpha (TNFα)) inhibit NPC differentiation by blocking the cell cycle via up-regulated expression of cell cycle–dependent kinase inhibitors (Pluchino et al., 2008). As undifferentiated cells, NPCs can thus produce a wide array of both secreted and transmembrane molecules which, in turn, exert immunomodulatory and neurotrophic functions leading to tissue repair (Irvin et al.
Brain Repair: The Role of Endogenous and Transplanted Neural Stem Cells
Introduction
The homeostatic regulatory role of endogenous NPCs
NPC transplantation as a therapeutic tool to promote brain repair
The bystander effect of transplanted NPCs
Stay updated, free articles. Join our Telegram channel
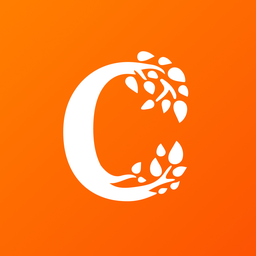
Full access? Get Clinical Tree
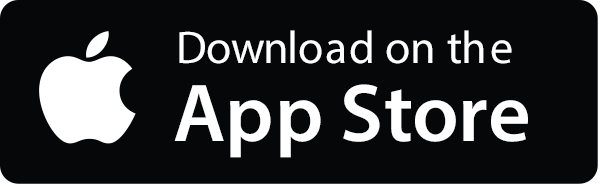
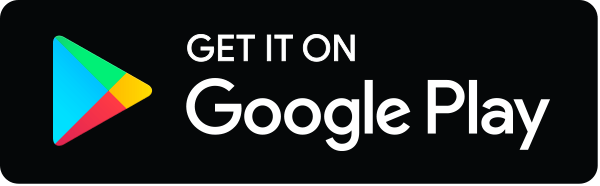