Breathing During Sleep: Ventilation and the Upper Airway
James A. Rowley
Safwan M. Badr
INTRODUCTION
The pathogenesis of sleep apnea syndrome remains poorly understood. The occurrence of apnea during sleep and not wakefulness implicates the removal of the wakefulness stimulus to breathe as a key factor underlying apnea and upper-airway obstruction during sleep. Development of sleep apnea or hypopnea involves an interaction between “host” risk factors and changes related to sleep state per se. Host risk factors could include gender, age, body mass index (BMI), and neck circumference, all of which have been associated with an increased prevalence of obstructive sleep apnea (OSA) (1,2,3 and 4). In this chapter, we address the fundamental effects of sleep on ventilation, upperairway patency, and the interaction with risk factors, such as gender and BMI. In addition, we discuss how sleep-related changes may conspire to induce recurrent apnea or upper-airway obstruction in susceptible individuals.
BREATHING DURING SLEEP: VENTILATION AND THE UPPER AIRWAY
Summary of Normal Breathing and Ventilation during Sleep
Nonrapid Eye Movement Sleep
The respiratory system changes during sleep to meet a decreased metabolic rate. Ventilatory motor output during sleep decreases from its normal levels in wakefulness, leading to decreased tidal volume and minute ventilation. The decreased ventilation is accompanied by reduced upper-airway dilator muscle activity resulting in decreased upper-airways caliber and increased airflow resistance. These biologic changes may account for the observed increase in partial arterial pressure of carbon dioxide (Paco2) and decrease in partial arterial pressure of oxygen (Pao2) during sleep, despite the diminished overall metabolism rate in the body. A decrease in chemoresponsiveness during sleep may also explain the increased Paco2. Overall, breathing becomes more dependent on chemical stimuli, which is integrated in the brainstem.
Rapid Eye Movement Sleep
In contrast to nonrapid eye movement (NREM) sleep, rapid eye movement (REM) sleep is characterized by variability in ventilation. This variability consists of sudden changes in respiratory amplitude and frequency associated with the periods of phasic REMs. Because of this variability, minute ventilation in REM sleep has been shown to be the same, increased, or decreased compared with NREM sleep. Upper-airway resistance has also been reported variably as either the same or increased compared to wakefulness and NREM sleep. Finally, hypercapnic and hypoxic ventilatory chemoresponsiveness is decreased in REM sleep compared to wakefulness and possibly even NREM sleep.
Effect of Sleep on Control of Breathing
Control of Breathing
Breathing is a rhythmic and automatic activity controlled by many mechanisms. The central nervous system (CNS) tightly controls the respiratory cycle. This regulation of breathing occurs at two different levels. The first level, the
brain cortex, allows humans to breathe voluntarily during wakefulness. The second level, the brain stem, regulates involuntary respiration, controlling it through many integrated responses between the peripheral nervous system and the CNS. Inspiration and expiration are under neural effect through groups of neurons located in the medulla, known as the respiratory center, which is divided into two separate sites: dorsal and ventral. The dorsal group is mainly responsible for the inspiration phase and the ventral site mainly maintains the expiratory phase. The spontaneous function of the respiratory center in the medulla is usually modified by other neurons in the pons, known as the pneumotaxic center. These neurons interact directly with mechanical receptors in the airways and lung. The CNS controls breathing not only through a mechanical feedback mechanism but also by chemical feedback coming from the blood.
brain cortex, allows humans to breathe voluntarily during wakefulness. The second level, the brain stem, regulates involuntary respiration, controlling it through many integrated responses between the peripheral nervous system and the CNS. Inspiration and expiration are under neural effect through groups of neurons located in the medulla, known as the respiratory center, which is divided into two separate sites: dorsal and ventral. The dorsal group is mainly responsible for the inspiration phase and the ventral site mainly maintains the expiratory phase. The spontaneous function of the respiratory center in the medulla is usually modified by other neurons in the pons, known as the pneumotaxic center. These neurons interact directly with mechanical receptors in the airways and lung. The CNS controls breathing not only through a mechanical feedback mechanism but also by chemical feedback coming from the blood.
Ventilation is the main function of breathing and considered a target of the breathing control mechanism. Ventilatory chemoreceptors are classified as either peripheral or central, depending on the anatomic location. These two types of receptors are highly integrated to respond to a change in Paco2, Pao2, or pH, which will influence the firing rate of specific respiratory motoneurons in the brainstem. In particular, central chemoreceptors are located on the ventral surface of medulla and are distinguishable from other respiratory centers. These chemoreceptors respond to changes in arterial Paco2 and the pH of the cerebrospinal fluid. Peripheral chemoreceptors are located in the carotid and aortic bodies. The peripheral chemoreceptors mainly sense the Pao2, in addition to Paco2 and pH, and send impulses to the medulla via the glossopharyngeal cranial nerve.
Ventilatory Control during Wakefulness and Sleep
Chemoresponsiveness refers to changing ventilation in response to changes in chemical stimuli. Chemosensitivity is influenced by changes in neural activity during sleep. Thus, hypoxic and hypercapnic chemoresponsiveness contributes to maintaining ventilation during sleep. Conversely, hypocapnia is a potent inhibitor of ventilation during NREM sleep and is a key mechanism of central apnea (5).
Hypercapnic Ventilatory Response
When arterial Paco2 rises, ventilation is stimulated linearly to bring the Paco2, level back to its normal range. Control of this response occurs as a central chemoresponse mainly in the ventral part of medulla. The sleep state is characterized by decreased hypercapnic ventilatory response (HCVR) in human adults compared to wakefulness (6,7,8,9 and 10). While the sensitivity to Paco2, does not appear to differ within NREM sleep stages, the HCVR during the REM stage is depressed further compared with NREM sleep (6,8).
Potential determinants of chemoresponsiveness during sleep include metabolic rate, age, gender, and the mechanics of the respiratory system. There is no empiric evidence of age effect on HCVR (11). Conversely, there are conflicting findings regarding the effect of gender on HCVR. Some have found no difference between the genders (8,9), whereas others have found a gender difference during wakefulness, but not sleep (6). One group found an increased HCVR during sleep in women compared with men (7). Thus, it is unclear whether there is a gender difference in HCVR.
The use of ventilation to express chemoresponsiveness requires that chemical stimuli do not alter upper-airway or pulmonary mechanics. Therefore, increased upper-airway resistance from wakefulness to NREM sleep would dampen ventilatory response to chemical stimuli and be interpreted as decreased chemoresponsiveness. The variability of the effect of changes in chemical stimuli on upper-airway caliber would further confound the interpretation of changes in chemoresponsiveness. Fortunately, recent studies have demonstrated that changes in the Paco2, and chemoresponsiveness occur independent of changes in upper-airway resistance (11,12). Nonetheless, the complex interaction between ventilation and upper-airway caliber mandates caution in interpreting sleep-related changes in chemoresponsiveness.
Hypoxic Ventilatory Response
Hypoxic ventilatory response (HVR) is the increase in minute ventilation in response to hypoxia. HVR is reported to decrease during NREM sleep compared to wakefulness, with a further decrease in REM sleep (10,13,14 and 15). Previous studies investigating the gender effect on HVR have come to conflicting conclusions. During sleep, men and women had similar HVR, although there was a large decrease in HVR from wakefulness in men, indicating that men had an increased HVR during wakefulness compared with women (13,14). Two recent studies have found similar responses to sustained isocapnic hypoxia between the two genders during wakefulness (16,17). In particular, we have recently demonstrated that the ventilatory response to brief hypoxia was similar between men and women in the absence of changes in upper-airway mechanics (17). This allowed us to conclude that there is no intrinsic gender difference in HVR during NREM sleep.
Effect of Sleep on Hypocapnic Ventilatory Response and the Apneic Threshold
The loss of wakefulness stimulus to breathe renders ventilation during NREM sleep critically dependent on chemoreceptor stimuli (Pao2 and Paco2). Reduced Paco2 is a powerful inhibitory factor of ventilation during sleep. Therefore, central apnea develops when Paco2 is reduced below a highly reproducible hypocapnic apneic threshold, unmasked by NREM sleep (5) (Fig. 4-1). Hypocapnia is probably the most important inhibitory factor during NREM sleep. Hypocapnia, secondary to hyperventilation, is key to the genesis of central sleep apnea in congestive heart failure (18) and idiopathic central sleep apnea (19,20).
Hypocapnia and unmasking of the apneic threshold may be relevant to the pathogenesis of OSA as well. First, by using fiberoptic imaging of the pharyngeal airway,
upper-airway obstruction in the absence of ventilatory motor output (central sleep apnea) has been observed (21) (Fig. 4-2). During hypocapnia-induced hypopneas, the reduction in cross-sectional area was observed specifically during the expiratory phase of the respiratory cycle, with no difference compared to control during inspiration (Fig. 4-3) (22). Second, changes in ventilatory motor output are associated with reciprocal changes in upper-airway resistance (23,24 and 25), especially in individuals with a highly collapsible airway such as snorers with inspiratory flow limitation (26). Furthermore, reduced ventilatory motor output lead to expiratory resistance is increased in the hypopneic breaths observed after induction of hypocapnia (22). Third, it has been shown that men are more susceptible to the development of central sleep apnea and have a decreased responsiveness to CO2 (27), a result consistent with the increased prevalence of OSA in men. Postmenopausal women also have an increased susceptibility to the development of central sleep apnea (28), a result consistent with the increased prevalence of OSA in postmenopausal women (29). The gender differences appear, at least in part, secondary to testosterone as there was no difference in apneic threshold between the follicular and luteal stages in women (27), and the administration of testosterone to premenopausal women results in an increased apneic threshold, similar to that seen in men (30).
upper-airway obstruction in the absence of ventilatory motor output (central sleep apnea) has been observed (21) (Fig. 4-2). During hypocapnia-induced hypopneas, the reduction in cross-sectional area was observed specifically during the expiratory phase of the respiratory cycle, with no difference compared to control during inspiration (Fig. 4-3) (22). Second, changes in ventilatory motor output are associated with reciprocal changes in upper-airway resistance (23,24 and 25), especially in individuals with a highly collapsible airway such as snorers with inspiratory flow limitation (26). Furthermore, reduced ventilatory motor output lead to expiratory resistance is increased in the hypopneic breaths observed after induction of hypocapnia (22). Third, it has been shown that men are more susceptible to the development of central sleep apnea and have a decreased responsiveness to CO2 (27), a result consistent with the increased prevalence of OSA in men. Postmenopausal women also have an increased susceptibility to the development of central sleep apnea (28), a result consistent with the increased prevalence of OSA in postmenopausal women (29). The gender differences appear, at least in part, secondary to testosterone as there was no difference in apneic threshold between the follicular and luteal stages in women (27), and the administration of testosterone to premenopausal women results in an increased apneic threshold, similar to that seen in men (30).
REM sleep is a special case because peripheral atonia is accompanied by augmented inspiratory medullary neuronal activity, and the REM sleep electroencephalogram (EEG) shares many features of the awake EEG. Whether hypocapnia inhibits ventilation during REM sleep is yet
to be established in humans. In dogs, central apnea following hyperventilation can be demonstrated during REM sleep, but no systematic relationship existed with the magnitude of hypocapnia (31).
to be established in humans. In dogs, central apnea following hyperventilation can be demonstrated during REM sleep, but no systematic relationship existed with the magnitude of hypocapnia (31).
Ventilatory Instability in Obstructive Sleep-Disordered Breathing
There is increasing evidence of ventilatory instability in patients with OSA, suggesting a mechanistic interaction between ventilatory instability and upper-airway anatomy. This evidence consists of both research elucidating the interaction between underlying mechanisms of sleep-disordered breathing (32,33,34 and 35) and clinical studies showing the emergence of central sleep apnea after therapeutic tracheostomy (36) or initiation of continuous positive airway pressure (37,38 and 39). Susceptibility to develop periodic breathing reflects the overall gain of the chemoreceptor control of ventilation, referred to as the “loop gain.” Loop gain is an engineering concept used to as a framework to express the overall ventilatory change for a given initial perturbation. Loop gain is determined by several factors (40,41,42 and 43). The first component is plant gain. This factor relates the level of Paco2 for a given VE on the metabolic hyperbola curve. In general, a higher plant gain (generally associated with a lower Paco2 for a given minute ventilation) is associated with increased susceptibility to instability. The second major component is the controller gain representing the ventilatory response to changes in Paco2. Increased controller gain (generally associated with a lower CO2 reserve or the change in Paco2 associated with the development of apnea) is a marker of increased instability. The final contributor to loop gain is the delay introduced by gas mixing and diffusion; this latter contributor is rarely measured in research practice.
By using proportional assist ventilation to measure ”loop gain” in patients with sleep apnea, Younes et al. (33) found higher loop gain in patients with severe disease compared to mild disease. Likewise, Wellman et al. (35) found a strong correlation between loop gain and apnea hypopnea index (AHI) in a subset of patients with OSA. Recently, Salloum et al. (44), by using mechanical ventilation to induce hypocapnic central apnea, compared plant and controller gain in subjects with and without sleep apnea. There was no difference in plant gain between the two groups, whereas the controller gain was increased and the CO2 reserve decreased in the subjects with OSA.
Despite the above-mentioned evidence, it is unclear whether the observed ventilatory instability underlies the development of sleep apnea or is secondary to untreated OSA. In support of the latter, Salloum et al. (44) found that 1 month of positive airway pressure therapy resulted in a decrease in controller gain and an increase in CO2 reserve in sleep apnea subjects. Loewen et al. (45) has also recently found an improvement in measures of ventilatory instability, particularly, in the dynamic response to hypoxia and hypercapnia, after positive airway pressure in subjects with sleep apnea. These results would indicate that the ventilatory instability associated with sleep apnea is secondary to the recurrent airway collapse and oxyhemoglobin desaturation.
Effect of Sleep on Upper-Airway Structure and Function
The sleep state is a challenge, rather than a rest period, for the ventilatory system. Consequences of loss of wakefulness include reduced activity of upper-airway dilators, reduced upper-airway caliber, increased upper-airway resistance, loss of load compensation, and increased pharyngeal compliance and collapsibility. Ultimately, these changes lead to reduced tidal volume and hypoventilation (Fig. 4-4).
Reduced Upper-Airway Dilating Muscle Activity
The musculature of the upper airway consists of 24 pairs of striated muscles extending from the nares to the larynx (46,47). There are at least 10 muscles that are classified as pharyngeal dilators, innervated by more than one cranial nerve motoneuron. There are two patterns of electrical
discharge from these muscles: tonic (constant) activity, independent of phase of respiration, and phasic activity, occurring during one part of the respiratory cycle. It is widely accepted that upper-airway narrowing during sleep is due to a sleep related decrease in upper-airway muscle activity However, data demonstrating reduced motor output to upper-airway muscles during NREM sleep remain fragmentary and based mostly on animal studies using reduced or chronically instrumented preparations. Orem et al. (48) have shown, in several studies in cats, that peak firing rate of motoneurons in the ventral respiratory group is reduced during NREM sleep. Recent work in naturally sleeping, chronically instrumented rats has shown reduced electromyographic (EMG) activity of the genioglossus in NREM and in REM sleep (49). Overall, the available data from animal studies taken together suggest a small, but perhaps significant, reduction in the ventilatory motor output to upper-airway muscles.
discharge from these muscles: tonic (constant) activity, independent of phase of respiration, and phasic activity, occurring during one part of the respiratory cycle. It is widely accepted that upper-airway narrowing during sleep is due to a sleep related decrease in upper-airway muscle activity However, data demonstrating reduced motor output to upper-airway muscles during NREM sleep remain fragmentary and based mostly on animal studies using reduced or chronically instrumented preparations. Orem et al. (48) have shown, in several studies in cats, that peak firing rate of motoneurons in the ventral respiratory group is reduced during NREM sleep. Recent work in naturally sleeping, chronically instrumented rats has shown reduced electromyographic (EMG) activity of the genioglossus in NREM and in REM sleep (49). Overall, the available data from animal studies taken together suggest a small, but perhaps significant, reduction in the ventilatory motor output to upper-airway muscles.
The effect of NREM sleep on upper-airway muscle function in humans has not been conclusively studied, partly due to the difficulty in isolating the myriad influences on upper-airway muscle activity, especially changes in flow or the magnitude of negative pressure in the pharyngeal airway. However, available evidence indicates a reduction in either the tonic or phasic activity during NREM sleep for a variety of upper-airway muscles (47), including the levator palatini (50), tensor palatini (51), palatoglossus (50), and geniohyoid (52).
The effect of REM sleep on upper-airway muscle activity is more compelling. Activity of antigravity muscles is reduced during REM sleep. There is strong evidence that activity of phasic upper-airway dilating muscles, such as the genioglossus, is greatly attenuated during REM sleep (53,54), particularly during periods of phasic REMs (55,56). Reduced activity has also been shown for the alae nasl (55) and geniohyoid muscles (52).
The response of upper-airway muscle to chemical and mechanical perturbations may be more relevant physiologically than reduced baseline activity. Pharyngeal muscles display an attenuated response to negative pressure during NREM (57,58 and 59) and REM sleep (60) compared to wakefulness. Similarly, responsiveness of the genioglossus muscle to hypercapnia is also attenuated during sleep (61). Decreased responsiveness to challenges indicates that upper-airway muscles are less able to maintain upper-airway patency in the face of chemical or mechanical perturbations. However, recent studies do not show a difference in genioglossus muscle activity between control subjects and subjects with OSA (62,63), indicating that upper-airway dilator activity during sleep may not be a predominant determinant of upper-airway collapse.
Upper-Airway Resistance
The evidence for increased upper-airway resistance during sleep is compelling, even in normal subjects (64,65,66,67 and 68). An example of increased upper-airway resistance during sleep is shown in Figure 4-5. In particular, Kay et al. (64) studied the changes in minute ventilation and upper-airway resistance during the transition from predominant alpha EEG activity through predominant delta activity. The group found that a large decrease in minute ventilation with sleep onset was associated with a small increase
in upper-airway resistance. In subjects who achieved delta activity, there was a progressive increase in upper-airways resistance that was not associated with further decreases in minute ventilation. The large increases in resistance were not observed in subjects who remained in stage 2 sleep during the study period. The preponderance of evidence is that there are no further increases in upper-airway resistance during REM sleep (67,68 and 69).
in upper-airway resistance. In subjects who achieved delta activity, there was a progressive increase in upper-airways resistance that was not associated with further decreases in minute ventilation. The large increases in resistance were not observed in subjects who remained in stage 2 sleep during the study period. The preponderance of evidence is that there are no further increases in upper-airway resistance during REM sleep (67,68 and 69).
Potential determinants of upper-airway resistance during sleep include many variables known to be associated with increased prevalence of OSA, such as gender, BMI, and age. Two groups of investigators have found no difference in upper-airway resistance between genders (69,70) during NREM, predominantly stage 2, sleep. In contrast, Trinder et al. (71) found that while there was no difference in upper-airway resistance during the transition from wakefulness to NREM sleep, there was an increased resistance in men during slow-wave sleep. Overall, there is no conclusive gender effect on upper-airway resistance during sleep.
The effect of age on upper-airway resistance is variable across different studies. Browne et al. (72) and Thurnheer et al. (69) found no difference in upper-airway resistance between young and older subjects. In contrast, we performed a linear regression analysis to determine the independent predictors of upper-airway resistance in a group of 60 subjects. For this group, age was the only independent predictor of upper-airway resistance, with an increased age associated with an increased upper-airway resistance. Interestingly, BMI was not a predictor of resistance (53). Recently, another group found that while upper-airway resistance was not different during wakefulness, there was a larger increase in resistance during sleep in older subjects compared to younger subjects (73). The reasons for the discrepancy of studies are not clear and may be due to subtle methodologic differences or to an interaction between age and other demographic factors in our study, such as the racial composition of the population. Nevertheless, the effect of age, per se, on upper-airway resistance appears modest even in our study.
It is important to note that upper-airway resistance provides only a partial picture of the dynamic behavior of the pharyngeal airway during sleep. Specifically, upper-airway resistance is generally expressed as a single number representing the slope of pressure—flow relationship during inspiration. This computation is predicated on a constant relationship between driving pressure and inspiratory flow, which is true during normal breathing in normal subjects. However, many subjects exhibit inspiratory flow limitation, in which the pressure-flow graph (Fig. 4-4) demonstrates a changing relationship culminating in complete dissociation between pressure and flow (pressure continues to decrease with no further increase in flow). Thus, the only physiologically meaningful measurement of resistance is the slope of the linear portion of the pressure—flow loop, which likely reflects upper-airway caliber at the narrowest point in the upper airway at the beginning of inspiration. The potential for pressure-flow dissociation mandates the use of other indices to properly characterize upper-airway structure and function during sleep.
The aforementioned discussion indicates that decreased upper-airway caliber during sleep is a physiologic phenomenon. However, a substantial decrease in upper-airway caliber may cause “fluttering” of the soft palate due to turbulent flow, producing the acoustic phenomenon known as snoring. The mechanical corollary of snoring is inspiratory flow limitation, which manifests as a plateau in flow, despite continued development of negative pressure. Figure 4-6 is an example of increased upper-airway resistance and flow limitation during sleep in a normal subject. This representative polygraphic segment
depicts reduced flow with flattening of the flow profile with snoring and progressive decrease in supraglottic pressure, terminating with an arousal. Recurrent episodes of increased resistance and inspiratory flow limitation lead to increased work on breathing, hypoventilation, frequent arousals from sleep, and subsequent excessive daytime sleepiness. In fact, some authors identify this constellation of findings as a distinct clinical entity referred to as the upper-airway resistance syndrome (74), under the rubric of sleep-related breathing disorders.
depicts reduced flow with flattening of the flow profile with snoring and progressive decrease in supraglottic pressure, terminating with an arousal. Recurrent episodes of increased resistance and inspiratory flow limitation lead to increased work on breathing, hypoventilation, frequent arousals from sleep, and subsequent excessive daytime sleepiness. In fact, some authors identify this constellation of findings as a distinct clinical entity referred to as the upper-airway resistance syndrome (74), under the rubric of sleep-related breathing disorders.
It is commonly assumed that snoring and flow limitation are tightly linked to increased upper-airway resistance. However, it is plausible that resistance would be high in a stiff, but narrow, tube. To evaluate such association, we evaluated BMI and upper-airway resistance as potential determinants of the presence of inspiratory flow limitation in >10% of breaths in a group of normal subjects (70). By using multivariate logistic regression, we determined that BMI and upper-airway resistance both predicted the presence of inspiratory flow limitation. Interestingly, while increased BMI was associated with an increased likelihood of inspiratory flow limitation, increased upper-airway resistance was associated with a decreased likelihood of inspiratory flow limitation, indicating that a narrow upper airway may be less susceptible to distortion by subatmospheric intrathoracic pressure. Upper-airway obstruction may be more common in individuals with a narrow upper airway because of the influence of structures and tissues that surround the upper airway, not because of the narrowed upper airway per se.
In addition, many authors equate increased upper-airway resistance to increased collapsibility However, our group recently found that the expected decrease in upper-airway resistance after recurrent hypoxia was not associated with a decrease in upper-airway collapsibility (75). Thus, upper-airway resistance during sleep is a rather limited surrogate for susceptibility to pharyngeal closure during sleep.
Reduced Upper-Airway Caliber
By using nasopharyngoscopy during naturally occurring sleep in normal subjects, Rowley et al. (67,68) have shown that pharyngeal cross-sectional area is decreased during sleep at both the retropalatal and retroglossal levels. During NREM sleep, both retropalatal cross-sectional area and retroglossal cross-sectional area decreased to approximately 70% of the awake baseline cross-sectional area. The decreased cross-sectional area is consistent with a decrease in upper-airway dilator activity with the onset of NREM sleep. In REM sleep, retropalatal cross-sectional area did not decrease further compared with NREM sleep (68). In contrast, retroglossal cross-sectional area did decrease further during REM compared with NREM sleep (67). The contrast between the influence of REM sleep on retropalatal and retroglossal area can be explained in two ways. First, there may not be a further decrease during REM sleep in the activity of the dilators that control the retropalatal area (including the levator and tensor palatini) in contrast to a demonstrated decrease in genioglossus activity during REM sleep. Second, it is possible that nonneuromuscular factors, such as the bony structures of the nasopharynx (including the pterygoid hamulus), could act to keep the airway open during periods of decreased neuromuscular activity.
The effect of gender on upper-airway cross-sectional area has been extensively investigated. The preponderance of studies indicates no difference in cross-sectional area between men and women during wakefulness (76,77 and 78). In contrast, by using fiberoptic nasopharyngoscopy, we have shown that the retropalatal cross-sectional area was smaller in women than men during NREM sleep. However, the absolute gender difference disappeared when cross-sectional area was corrected for body surface area. In addition, sleep-related narrowing occurred to a similar degree in men and in women (˜ a 40% decrease in cross-sectional area for both genders) between wakefulness and NREM sleep (79).
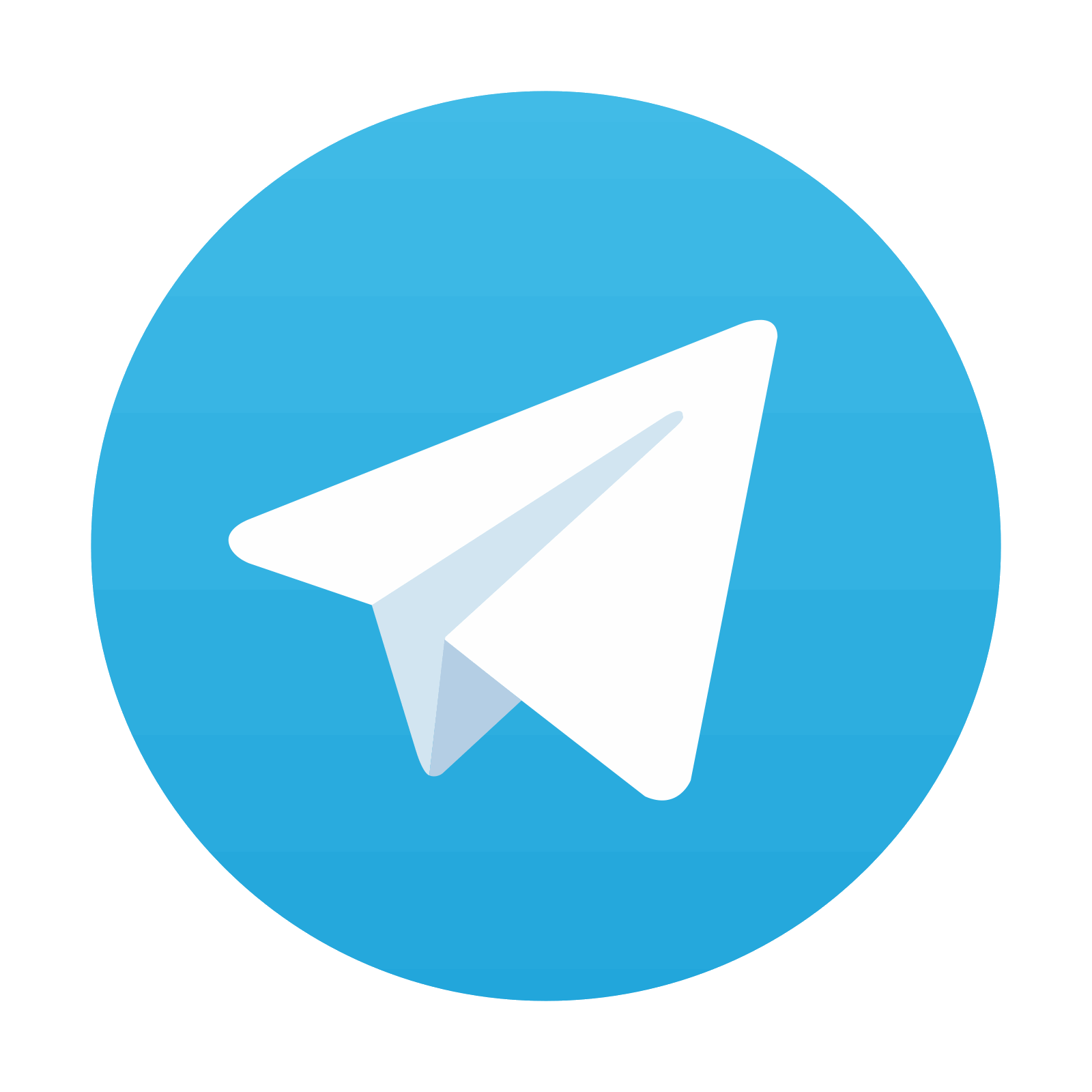
Stay updated, free articles. Join our Telegram channel
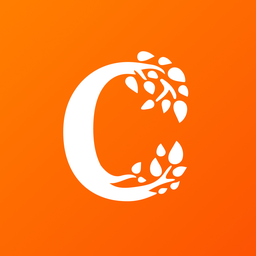
Full access? Get Clinical Tree
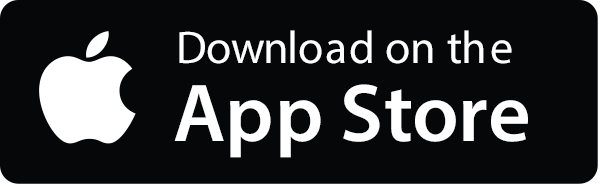
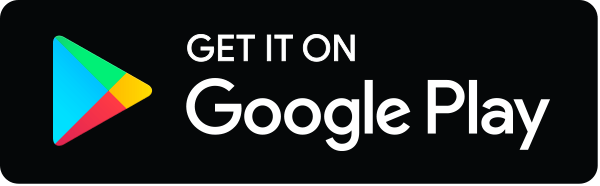
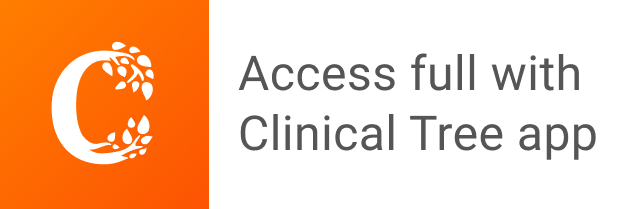