Cardiovascular complications after brain injury
Brain disorders including traumatic brain injury (TBI) have been known to affect the heart directly or indirectly. These conditions may include cardiomyopathy, arrhythmias, or autonomic dysfunction.
Cardiomyopathy
Cardiomyopathies caused by brain injury have been attributed to catecholamine and neuroendocrine excess and as a result of the inflammatory process. There are two described syndromes: Takotsubo cardiomyopathy (TCM) and neurogenic stunned myocardium (NSM). Both share similar mechanisms and have similar clinical manifestations and clinical courses. Some investigators feel that the two syndromes are in fact the same condition.
TCM has also been called left ventricular apical ballooning syndrome and broken heart syndrome . Its first investigators noted the left ventricle’s similar appearance to a Takotsubo or “octopus pot” used by Japan fishermen that has a round bottom and narrow neck. Since its initial identification in 1983, it has been seen worldwide in up to 2% of initially presumed acute coronary syndromes.
The Mayo criteria for TCM include:
- •
Hypokinesis, akinesis, or dyskinesis of the left ventricular midsegments with or without apical involvement
- •
Absence of obstructive coronary disease or acutely ruptured plaques
- •
Presence of new electrocardiogram (ECG) abnormalities (either ST-segment elevation or T-wave inversion)
- •
Absence of pheochromocytoma or myocarditis
NSM has a very similar presentation to TCM, with the main difference being a global hypokinesis of the left ventricle in NSM rather than the segmental wall dysfunction of TCM. Both TCM and NSM may be difficult to distinguish from acute coronary syndrome (ACS). However, both cardiomyopathy syndromes are notable for wall motions that do not correspond to ischemic ECG changes and troponin levels that are only mildly elevated (<2.8 ng/mL). These findings in patients without known heart disease or in new cardiac dysfunction (ejection fraction <40%) suggests an injury-induced cardiomyopathy over ACS.
Causative mechanisms of TCM and NSM may include transient coronary vasospasm, microvascular dysfunction, or myocyte injury caused by excess of catecholamines. It has been observed in association with several stressful settings, including sudden emotional stress or acute medical illnesses such as asthma. The proposed excess catecholamine mechanism has helped explain the appearance of this syndrome after acute brain syndromes such as stroke, ruptured cerebral aneurysm, seizures, encephalitis, or TBI.
The brain’s influence on the heart in this condition stems from damage to the insular cortex and subcortical regions. These areas have central influences on cardiovascular function and the autonomic nervous system. This leads to excess catecholamine release from sympathetic nerve endings in myocardium and autonomic dysfunction and neuroinflammation. The catecholamine release subsequently leads to intracellular calcium elevation and cyclic adenosine monophosphate (cAMP) overload with depletion of adenosine triphosphate (ATP) in the myocyte. The calcium influx also leads to myocardial reperfusion injury. Subsequent mitochondrial dysfunction and cell death ensues. Elevation of circulating catecholamines may last up to 10 days after injury, resulting in a persistent high sympathetic tone.
The patient with TCM typically presents with hypertension from the catecholamine storming followed by hypotension from the vasodilation and cardiac dysfunction. There is no agreed-upon management, and it is guided by the maintenance of normal hemodynamics and cerebral perfusion. Inotropic support with dobutamine will improve cardiac function. Beta blockers in the setting of TBI may be protective, because exposure to this medication class during acute hospitalization has demonstrated better outcomes, including reduced mortality. Generally, the effects of maintaining normal hemodynamics in the early stages will result in excellent cardiac outcomes with return of left ventricle function to normal within several weeks to months.
Arrhythmia
Several arrhythmias have been observed during the first week of brain injury, including sinus tachycardia, atrial fibrillation, premature atrial contractions, premature ventricular contractions, and atrioventricular (AV) dissociation. More severe arrythmias such as ventricular fibrillation are rarer and more commonly seen with cardiac injury. Injury to the insula cortex has been associated with prolongation of the QT interval See Table 18.1 . A prolonged QT interval has been associated with the dangerous arrhythmia torsades de pointes, and therefore medications known to prolong the QTc should be avoided in the acute hospital and perhaps into rehabilitation hospitalization. Medications known to prolong QTc include certain antiarrhythmics, antipsychotics, antidepressants and erythromycins. ,
Pharmacologic Class | Representative Examples |
---|---|
Acetylcholinesterase inhibitors | Donepezil |
Analgesics | Methadone |
Anesthetics | Propofol, desflurane, isoflurane, sevoflurane |
Antibacterials | Azithromycin, clarithromycin, clindamycin, erythromycin, roxithromycin, spiramycin, telithromycin, bedaquiline, ciprofloxacin, gatifloxacin, gemifloxacin, grepafloxacin, levofloxacin, moxifloxacin, ofloxacin, sparfloxacin |
Anticholinergic | Tolterodine |
Antidepressants | Amitriptyline, nortriptyline, desipramine, citalopram, fluoxetine, venlafaxine, trazodone |
Antifungals | Fluconazole, ketoconazole, posaconazole, voriconazole |
Antihistamines | Diphenhydramine, hydroxyzine, mizolastine |
Antimalarials | Quinidine, quinine, chloroquine, halofantrine, piperaquine |
Antineoplastic | Arsenic trioxide, depsipeptide, vorinostat, ivosidenib, lenvatinib, vandetanib |
Antivirals | Atazanavir, nelfinavir, saquinavir, efavirenz, foscarnet |
Antiarrhythmics | Amiodarone, disopyramide, dofetilide, procainamide, quinidine, sotalol |
Neuroleptics | Chlorpromazine, droperidol, haloperidol, pimozide, quetiapine, risperidone, sertindole, thioridazine, ziprasidone, clozapine, olanzapine |
Promotility agents and antiemetics | Cisapride, dolasetron, domperidone, ondansetron, metoclopramide |
Vasodilators | Cilostazol |
Autonomic dysfunction
Survivors of TBI have been known to demonstrate alterations in autonomic functions, known as dysautonomia, after injury to the autonomic nervous system (ANS). Such injuries upset the normal homeostasis of the sympathetic and parasympathetic divisions of the ANS and lead to abnormalities in cardiac function, blood pressure, heart rate variability, gastrointestinal motility, pupillary contraction, and sphincter control. These alterations have been seen in diffuse axonal injury and with secondary injury from hypoxia and focal injuries to the insula, hypothalamus, and brainstem.
Following acute trauma, there is an increase in sympathetic activity with or without brain injury, but these effects are magnified in many cases of severe TBI. Initial responses include tachycardia, tachypnea, and hypertension, which are seen to some degree in most TBI patients in the intensive care unit (ICU). The release of excess catecholamines after injury appears to be responsible for this activity. In the most severe cases, a state of paroxysmal sympathetic hyperactivity (PSH) will emerge, in which extreme autonomic instability is combined with excess upper motor neuron motor activity, which will be discussed in detail in Chapter 24 .
Common cardiopulmonary effects associated with PSH include prolonged hypertension, arrhythmias, and tachypnea. Sympathetic overactivity may be demonstrated by heart rate variability in response to noxious stimuli. Persistent hypertension increases the risk of secondary brain injury through cerebral edema or expansion of hemorrhage. Additionally, prolonged hypertension places the patient at risk for cardiac dysfunction and resulting anoxic injury. Common arrhythmias during PSH include supraventricular tachycardia, ectopic beats, and atrial fibrillation.
Dysautonomia may also be seen in the rehabilitation admission or later. Autonomic instability may result in postural orthostatic tachycardia syndrome (POTS), which may be severe enough to cause syncope caused by impairment affecting the baroreflex system. Symptoms include palpitations, dizziness, and fatigue. Sympathetic outflow to afferent input from vagal nerve and carotid bodies is poorly modulated, leading to baroreceptor failure. Treatment includes medications to support blood pressure, which may include fludrocortisone, desmopressin, or midodrine. Selective serotonin reuptake inhibitors (SSRIs) or norepinephrine reuptake inhibitors have also been useful in cardiogenic syncope and may be considered in TBI patients with POTS. Tilt table training in physical therapy should be prescribed. Compression stockings and increased salt intake has also been advised.
Whereas autonomic dysfunction has been mostly associated with severe TBI, there is an increased recognition of abnormalities after concussion or mild TBI. Abnormalities described in this setting include heart rate variability as recorded on a 24-hour Holter monitor, changes in pupillary light reflex, eyeball pressure, and arterial pulse waves. Such symptoms when persistent may require delay in return-to-play protocols in athletes with concussion.
Pulmonary complications after brain injury
Patients with severe TBI may face several pulmonary complications in acute hospital management and extending into rehabilitation: pneumonia, pulmonary embolism, aspiration, and atelectasis. Many patients will have concomitant chest trauma, placing them at additional risk. Some severely injured patients may obtain tracheostomy tubes to allow for better airway protection, but this will require decisions about management, including decannulation during the rehabilitation phase.
Pneumonia
Pneumonia is a common early complication of severe TBI, with an incidence ranging up to 47%, often related to aspiration at the time of injury or associated with mechanical ventilation. Risk factors for an early pneumonia in severe brain injury include nasogastric tube insertion, hemiplegia or hemiparesis, and increased age. Polytrauma, whether caused by additional chest injuries or involvement of other body organs, has been cited as an additional risk factor for ventilator-associated pneumonia (VAP). Early identification of pneumonia is important to prevent secondary brain injury from fever, hypoxemia, or hypercapnia. Furthermore, there is a potential for critically ill patients with pneumonia to develop more severe inflammatory lung conditions including acute lung injury (ALI) or acute respiratory distress syndrome (ARDS). VAP in severe TBI patients has been associated with longer duration of mechanical ventilation, longer ICU length of stay, and higher incidence of tracheostomy placement.
Hospital-acquired pneumonia (HAP) has been cited as an independent predictor of poor global outcome up to 5 years after discharge after a severe TBI according to the TBI Model System outcome data. Lower Glasgow Outcome Scale-Extended (GOS-E) scores were noted in the patients who had acquired HAP (42 patients, mean GOS-E 5) versus those without HAP (99 patients, mean GOS-E 7) at 2 and 5 years postinjury. The cause of the extended effect of pneumonia could not be ascertained in this study because of data limitations, but chronic inflammation and secondary brain injury from hypoxemia were postulated.
Long-term studies have shown that long-term survivors of TBI face a higher risk of aspiration pneumonia and subsequent mortality risk. A large multicenter study revealed that TBI survivors discharged from rehabilitation units were 79 times more likely to die of aspiration pneumonia than the general population. Those with higher risks included persons with more severe disability and those discharged to nursing homes. Additional risk factors were prior aspiration pneumonia, percutaneous endoscopic gastrostomy (PEG) placement during acute or rehabilitation hospital admission, and persistent dysphagia at the time of rehabilitation discharge. A study of TBI mortality of TBI survivors up to 40 years after injury showed that patients were 1.5 times more likely to die of any cause, 4 times more likely to die of pneumonia, and 49 times more likely to die specifically from aspiration pneumonia compared with the general population. Higher mortality was associated with increased age at the time of injury, male sex, lower education level, longer hospital length of stay, and vegetative state.
Tracheostomy tubes
In severe TBI, endotracheal intubation is often required for airway protection to prevent hypoxia and secondary brain injury. Subsequently tracheostomy tube placement is often performed for patients needing extended ventilator support. In this setting a tracheostomy tube may improve patient comfort and pulmonary hygiene and reduce VAP and the need for sedation. Tracheostomy placement has been associated with improvement in survival rates. Risk factors for obtaining a tracheostomy tube after TBI include failed extubation and multiple operating room (OR) visits. Placement traditionally has been performed after 10 to 14 days after injury, but it has also been advocated earlier. Traditional tracheostomy placement has been associated with longer ICU stays and prolonged mechanical ventilation. However, earlier tracheostomy placement (<10 days) has resulted in reduced duration of mechanical ventilation, reduced incidence of pneumonia, and reduced length of ICU and total hospital days when compared with extended endotracheal intubation. , Tracheostomy placement is also associated with complications including wound infections, subcutaneous emphysema, esophageal injury, laryngeal nerve injury, and tracheal stenosis. So despite attempts to create protocols for tracheostomy placement, the decision for the procedure ultimately remains with the trauma surgeon.
Following release from the ventilator, decannulation can be considered and when appropriate will facilitate communication and swallowing function. Factors to consider before proceeding with decannulation may include the patient’s respirations, tracheal secretions, cough, phonation, and swallowing. Maximal expiratory pressure, spontaneous cough, and cough strength are positive predictors of tracheostomy weaning, whereas excessive secretions and anoxic brain injury have been associated with failures. Glasgow Coma Score (GCS) is not associated with failures, and decannulation can be performed successfully in vegetative patients when a strong cough is present. ,
There are a number of techniques and protocols to progress through the process of decannulation. Typical steps and options during the weaning process include:
- •
Deflation of the tracheostomy cuff
- •
Downsizing to a smaller diameter tracheostomy tube
- •
Replacement with a tube without a cuff when positive pressure respiratory treatments are no longer necessary
- •
Use of a speaking valve when the cuffed tube is in a deflated position
- •
Capping a cuffless tracheostomy tube for progressively longer hours of the day while monitoring oxygen saturations and vital signs
- •
Performing laryngoscopy on patients in whom there is suspicion of subglottic airway stenosis or tracheal granulation
- •
Tolerance of a closed tracheostomy tube for 72 hours is associated with successful decannulation
Venous thromboembolic prophylaxis
Deep venous thrombosis (DVT) and pulmonary embolism (PE) can be seen in up to 20% of patients with severe TBI who do not receive prophylaxis. This high incidence is caused by a combination of immobility, prolonged ventilation, and hypercoagulability. Mechanical and pharmacological interventions have been used to prevent these complications. There is no doubt that pharmacologic chemoprophylaxis reduces the incidence of venous thromboembolic (VTE) complications, but the difficult decision has been the timing of the initiation of medications, which can increase the risk of intracranial bleeding or expand existing hemorrhages. Delayed initiation of chemoprophylaxis beyond 4 days has been associated with a threefold increase in DVT, however, whereas a literature review determined that the rate of DVT climbed from 2.6% if the chemoprophylaxis was started by day 3 postinjury up to 14.1% if it was delayed to day 8.
The use of mechanical prophylaxis is safe for nearly all TBI patients and should be initiated early within 24 hours after TBI or within 24 hours of a craniotomy, but the evidence is considered low quality and is a weak recommendation of the Neurocritical Care Society.
The timing of safe initiation of chemoprophylaxis with either low-molecular-weight heparin (LMWH) or unfractionated heparin (UFH) has been studied in the setting of acute brain injury. Current practice by the American College of Surgeons recommends following the Modified Berne-Norwood Criteria See Table 18.2 . In these guidelines, it is safe to initiate chemoprophylaxis if there is a stable head computed tomography (CT) scan at 24 hours without hemorrhage—considered the low-risk group. Moderate-risk patients may have pharmacologic prophylaxis at 72 hours if hemorrhage on earlier CT images is stable. In high-risk patients (those with intracranial pressure [ICP] monitor placement, craniotomy, or with further evidence of hemorrhagic progression on head CT at 72 hours), placement of a retrievable inferior vena cava (IVC) filter should be considered.
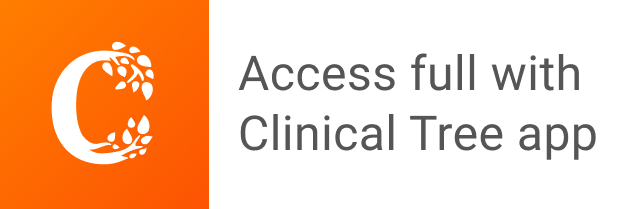