Fig. 2.1
Cellular and molecular effects of repetitive transcranial magnetic stimulation (rTMS) relevant to neural plasticity. (a) Schematic illustrating the effects of rTMS on neural tissue. While experimental evidence has been provided that single-pulse TMS can elicit action potentials, the role of structural and functional properties of distinct neurons and local circuitries (e.g., recurrent networks, feed-forward, and feed-back inhibition) remains not well understood. In this context input-/synapse-specific effects (CB calbindin; PV parvalbumin) and TMS effects on non-neuronal cell types, i.e., glial (astrocytes, oligodendrocytes, microglia), endothelial, and immune cells, must be considered as well. It has become clear that rTMS can change structural, functional, and molecular properties of neurons, which may depend on the simultaneous induction of both anterograde and backward propagating action potentials. Neuromodulation is expected to play a fundamental role in this context. However, the precise role of rTMS in promoting, blocking, or shifting the balance between distinct forms of plasticity remains to be determined. (b, c) Illustration of potential direct or indirect molecular targets of rTMS. (b) Experimental evidence suggests that rTMS-induced plasticity requires the activation of voltage-gated sodium channels (VGSCs), N-methyl-D-aspartate receptors (NMDARs), and L-type voltage-gated calcium channels (L-VGCCs) during stimulation. The induced changes in excitatory synaptic strength (long-term potentiation/depression, respectively; LTP/LTD) are linked to the molecular reorganization of dendritic spines and postsynaptic densities (PSD95), including the phosphorylation of α-amino-3-hydroxy-5-methyl-4-isoxazolepropionic acid receptor (AMPAR) and changes in synaptic AMPAR content. An involvement of presynaptic mechanisms (VGlut1; vesicular glutamate transporter 1), metabotropic neurotransmission (mGluR; metabotropic glutamate receptors and its anchoring protein Homer 1a), and remodeling of the cytoskeleton have been reported in this context as well. (c) While the precise intracellular signaling pathways of rTMS-induced plasticity remain not well understood, brain-derived neurotrophic factor (BDNF) and cyclic adenosine monophosphate (cAMP)-dependent signaling pathways have been identified to play an important role. These and other pathways could be involved in rTMS-mediated changes in gene expression profiles and proteostasis
While a wealth of information has been acquired on the cellular and molecular mechanisms of various forms of plasticity under physiological conditions, the interplay between distinct forms of plasticity (e.g., Hebbian plasticity, homeostatic plasticity, metaplasticity; see Table 2.1) and their role for neurological and psychiatric diseases remains not well understood (Maggio and Vlachos 2014). Recent evidence suggests that the ability of neurons to express plasticity may change and/or plasticity mechanisms may be recruited in a nonspecific manner under pathological conditions (Hulme and Jones 2013). It has become clear that an impairment of plasticity cannot be simply interpreted as detrimental under pathological conditions, since a reduction in the ability of neurons to express plasticity may protect the brain from “maladaptive changes”, which promote the development of disease-related complications such as epilepsy, pain, or memory dysfunction (e.g., Ferguson et al. 2012; Leuner and Shors 2013; Moxon et al. 2014; Nava and Röder 2011; Papa et al. 2014; Swann and Rho 2014; Winkelmann et al. 2014; Zenonos and Richardson 2014). Thus, with a better understanding on the role of neural plasticity under pathological conditions, novel therapeutic approaches could be designed to promote, block, or shift the balance between distinct forms of plasticity in specific brain regions and at diverse stages of pathological brain conditions (Maggio and Vlachos 2014).
Table 2.1
Major forms of neural plasticity
Form of plasticity | Short summary/definition |
---|---|
Hebbian plasticity | Named after Donald Hebb (1904–1985), this form of associative plasticity, in which simultaneous or rapid sequential activation of two synaptically connected neurons leads to a change in the strength of synapses between them (James 1890), describes structural, functional, and molecular adaptations of neurons that are considered to underlie experience-dependent network changes, as seen in the context of learning and memory. A classic experimental approach to study this form of plasticity is electrical induction of long-term potentiation (LTP; Bliss and Lomo 1973). The discovery of spike timing-dependent plasticity (Markram et al. 1997; Bi and Poo 1998; Song et al. 2000) supported the temporal causality proposed by Hebb (i.e., “cell A firing cell B,” Hebb 1949) to play an important role in promoting specific changes in network connectivity. |
Homeostatic plasticity | Describes compensatory mechanisms, which promote stability of neural networks despite ongoing (experience-dependent) changes (Davis 2006; Marder and Goaillard 2006; Turrigiano 2008; Pozo and Goda 2010). Involves the modification of intrinsic, synaptic, and structural properties of neurons that aim at keeping functionality in neural networks within a proper dynamic range. If, for example, network activity increases, neurons will respond after a while with a compensatory reduction in excitatory synaptic strength (or an increase in inhibitory synaptic strength). |
Metaplasticity | Subsumes mechanisms, which regulate the duration, direction, and extent of associative plasticity, without directly affecting neural excitability, transmission, and connectivity (Abraham and Bear 1996). This form of plasticity controls the ability of neural networks to express plasticity (“plasticity of plasticity”). |
Repetitive transcranial magnetic stimulation (rTMS) represents an interesting diagnostic and therapeutic tool in this context. Although our understanding on the cellular and molecular mechanisms underlying rTMS-based therapies remains limited (Müller-Dahlhaus and Vlachos 2013), it has been demonstrated that repetitive magnetic stimulation (rMS) is capable of recruiting plasticity-related mechanisms in neural tissue.
2.2 The Effects of rTMS During Stimulation
Using computational approaches to estimate cortical electric fields induced by TMS in combination with simulations of the effects of electric fields on neurons, some insights into TMS effects on neural tissue have been gained (e.g., Basser 1994; Opitz et al. 2011; Rotem and Moses 2008; Rusu et al. 2014). Nevertheless, it has remained largely unknown how TMS affects individual neurons within distinct cortical networks (Dayan et al. 2013).
A major limitation in this field of research has been the challenge to record from individual neurons during stimulation, due to the strong electromagnetic field induced by TMS. Recent technical advances, however, have made it possible to assess neural activity during stimulation using electrophysiological (Muller et al. 2014; Pashut et al. 2014) or functional optical imaging techniques (Kozyrev et al. 2014). These studies provide experimental evidence that single-pulse magnetic stimulation initiates action potentials preferentially in low-threshold interneurons (Pashut et al. 2014), resulting in a suppression of the stimulated cortex for about 200 ms after stimulation (Kozyrev et al. 2014). Conversely, high-frequency repetitive magnetic stimulation (10 Hz; or single-pulse stimulation with higher intensity) seems to shift the balance between excitation and inhibition toward excitation (Kozyrev et al. 2014). Additional work is now required to better understand how structural and functional properties of individual neurons and specific network architectures influence the outcome of single-pulse and repetitive magnetic stimulation.
In this context, recent work has also indicate that rMS may assert its effects by simultaneously depolarizing pre- and postsynaptic neuronal compartments, i.e., through the induction of both anterograde and backward propagating action potentials (Lenz et al. 2015). Hence, simultaneous recordings of distinct cells or dual recordings from individual neurons, e.g., somato-dendritic recordings, are expected to provide new important insights into the effects of rTMS during stimulation at the single-cell level. The impact of rTMS on non-neuronal cell types in the brain (e.g., astrocytes, microglia, oligodendrocytes, endothelial cells, immune cells) remains to be determined (Fig. 2.1a).
2.3 Repetitive Magnetic Stimulation Induces Plasticity of Excitatory Synapses
Early reports in human subjects have demonstrated that rTMS can increase or decrease cortical excitability beyond the stimulation period (Chen et al. 1997; Ziemann et al. 2008). It was noted that stimulus intensity, frequency, and the state of the stimulated network influence the duration, direction, and extent of rTMS-induced changes in cortical activity (for details, see Chap. 1). These after-effects of rTMS have been assumed to represent changes in synaptic efficacy and were therefore termed “long-term potentiation and depression (LTP/LTD)-like” phenomena, respectively. Hence, it was proposed that rTMS could assert its beneficial effects in the context of neurological and psychiatric disease by interfering with Hebbian forms of plasticity, e.g., LTP/LTD, which is considered to underlie learning and memory processes. Accordingly, animal studies have been employed to assess the effects of rTMS on synaptic plasticity. Initial experimental evidence for rTMS-induced synaptic activity was derived from immunostainings for immediate early gene (IEG)-encoded proteins, such as c-fos and zif-268 (e.g., Barth 2007; Loebrich and Nedivi 2009; Okuno 2011; Smeyne et al. 1992), which are recruited in the early stage of synaptic plasticity. Although robust experimental evidence has been provided that rTMS recruits IEG-encoded proteins, increased levels of c-fos and zif-268 were observed independent of stimulation frequency and pattern (Aydin-Abidin et al. 2008; Hausmann et al. 2000, 2001; Hoppenrath and Funke 2013; Volz et al. 2013). Yet, it was noted that rTMS may activate distinct brain regions and specific neurons within stimulated networks (Ji et al. 1998). Likewise, immunostainings for presynaptic (Vlachos et al. 2012; Volz et al. 2013) and postsynaptic markers (Gersner et al. 2011; Lenz et al. 2015; Ma et al. 2013; Vlachos et al. 2012; Fig. 2.1b) provided evidence that synaptic changes may underlie rMS-induced plasticity. More recent work in organotypic slice cultures was able to provide direct experimental evidence at the single-cell level that rMS is capable of inducing long-lasting functional and structural synaptic plasticity, that is an N-methyl-D-aspartate receptor (NMDAR)-dependent, Ca2+-mediated enlargement of dendritic spines and strengthening of excitatory synapses (Vlachos et al. 2012; Lenz et al. 2015). These studies are in line with earlier in vivo and in vitro work (e.g., Levkovitz et al. 1999; Tokay et al. 2009) supporting the notion that rTMS of the human cortex may induce Hebbian-type synaptic plasticity, i.e., LTP of excitatory synapses.
Although rMS has been shown (1) to require the activation of voltage-gated sodium channels (VGSC); (2) to be Ca2+-dependent, i.e., requiring the activation of both NMDAR and L-type voltage-gated calcium channels (L-VGCC) (Vlachos et al. 2012; Lenz et al. 2015); (3) to recruit intracellular signals such as cAMP-CREB (Hellmann et al. 2012); and (4) to depend on BDNF-TrkB signaling (Fig. 2.1c; Wang et al. 2011; Ma et al. 2013), the precise downstream signaling pathways leading to LTP of excitatory synapses following rTMS, such as phosphorylation and/or accumulation of α-amino-3-hydroxy-5-methyl-4-isoxazolepropionic acid receptors (AMPAR) at excitatory postsynapses (Gersner et al. 2011; Vlachos et al. 2012; Lenz et al. 2015), warrant further investigation (Fig. 2.1b, c). Future studies employing (opto-)genetic, pharmacologic, computational, and other experimental approaches will help in delineating similarities and differences between LTP mechanisms recruited by electromagnetic vs. local electric stimulation and may help in defining specific stimulation parameters for the effective induction of structural, functional, and molecular plasticity of distinct synapses in defined cortical networks by rTMS.
2.4 Repetitive Magnetic Stimulation Affects Inhibition and Neuronal Excitability
In addition to its effects on excitatory synapses, rTMS is expected to also modulate inhibitory neurotransmission. A variety of activity markers and calcium-binding proteins of inhibitory interneurons have been assessed in this context (e.g., Labedi et al. 2014; Mix et al. 2014, 2015; Trippe et al. 2009). For instance, it has been shown that intermittent theta-burst stimulation reduces parvalbumin (PV)-expression in fast-spiking interneurons, while continuous theta-burst stimulation and 1 Hz rTMS predominantly affect calbindin (CB)-expression in cortical areas (Benali et al. 2011; Trippe et al. 2009; Volz et al. 2013). As PV-expressing interneurons primarily control pyramidal cell output, i.e., somatic inhibition, whereas CB-expressing interneurons are considered to regulate pyramidal cell input, i.e., dendritic inhibition (c.f., Fig. 2.1a), these findings imply that distinct rTMS protocols may affect specific aspects of inhibition and hence network activity and function (Funke and Benali 2011; see also Mix et al. 2014, 2015). In line with this notion, TMS-EEG experiments in humans demonstrate that GABAergic inhibitory neurotransmission has a major impact on cortical excitability and connectivity (Premoli et al. 2014). However, direct experimental evidence for the effects of rTMS on inhibition is still missing, since to date no studies are available assessing rTMS-induced structural and functional changes of GABAergic synapses on principle neurons (or excitatory synapses on inhibitory interneurons). Similarly, a comprehensive analysis of rTMS effects on passive and active intrinsic cellular properties, e.g., voltage-gated sodium, potassium, chloride, and calcium currents, is required to better understand the effects of rTMS on excitation and inhibition (E/I) balance in neural circuits and their relevance for plasticity.
2.5 The Role of rTMS-induced Structural Plasticity in Modulating Network Connectivity
Structural changes, such as axonal sprouting and pruning, remodeling of the dendritic tree, dendritic spine turnover, and the formation or loss of excitatory and inhibitory synapses, continuously modify connectivity in the CNS. Since structural plasticity is known to depend on neural activity, it is conceivable that rTMS could assert long-lasting effects on neural networks by inducing the structural remodeling of neural networks. However, so far only one published study exists, which has employed in vitro live-cell microscopy to assess the dynamics of rMS-induced structural plasticity (Vlachos et al. 2012). In this study an increase in the volume of dendritic spines was reported to occur predominantly in small spines, while no effects on spine numbers were observed after high-frequency (10Hz) rMS (Vlachos et al. 2012). These findings are consistent with recent data on spine densities obtained from fixed tissue in vivo (Sykes et al. 2013). Since synapses on small spines are known to constitute weak synapses with low numbers or even no AMPARs (so-called silent synapses containing mainly NMDARs; e.g., Hanse et al. 2013; Kerchner and Nicoll 2008), it is possible that rTMS could modulate network connectivity by recruiting these weak or silent synapses without the need of additional spino- or synaptogenesis. It is tempting to speculate that a simultaneous depolarization of pre- and postsynaptic compartments, i.e., rTMS-induced anterograde (aAP) and backward propagating action potentials (bAP), may recruit silent synapses by increasing the probability of presynaptically released glutamate to activate postsynaptic NMDARs in the absence of AMPARs (see “bAP-aAP theory” in Lenz et al. 2015). Apparently, more work is required to clarify the contribution of “synaptic unsilencing” in rTMS-induced plasticity (see also Rodger et al. 2012) and to determine the effect of single vs. repeated rTMS sessions on structural properties (i.e., axons, dendrites, spines, synapses) of individual neurons, and other cells in the CNS.
2.6 Repetitive Magnetic Stimulation Modulates Gene Expression Profiles
Experimental evidence indicates that rTMS can modify gene expression profiles relevant for neural plasticity (Müller et al. 2000; Stock et al. 2012; Okada et al. 2002). However, it remains to be shown how rTMS-induced changes in gene expression affect proteostasis (i.e., the balance between biogenesis, folding, trafficking, and degradation of specific proteins; for review on proteostasis, see, e.g., Mardones et al. 2014), in distinct neural compartments, and how the observed effects influence the ability of neurons to express plasticity (Fig. 2.1). Neuroprotective, e.g., expression of neurotrophic factors such as BDNF (e.g., Gersner et al. 2011), but also toxic effects (Fang et al. 2010; Fujiki and Steward 1997; Okada et al. 2002) of rTMS must be considered in this context as well.
2.7 The Role of Neuromodulators in rTMS-induced Plasticity
Neuromodulation is another relevant aspect to consider in the context of rTMS-induced plasticity (e.g., Vahabzadeh-Hagh et al. 2012). It is plausible that dopamine, serotonin, acetylcholine, adrenaline, and other neuromodulators may affect the outcome of rTMS. In turn, it is possible that rTMS may act on these neuromodulatory systems to influence plastic properties of neuronal networks beyond the stimulation period.
Indeed, human studies disclose that rTMS-induced LTP- and LTD-like plasticity in the primary motor cortex depends on neuromodulation (Korchounov and Ziemann 2011; Thirugnanasambandam et al. 2011; for review, see Ziemann et al. 2015). Similarly, alterations in rTMS-induced motor cortex plasticity were reported in a rat model of Parkinson’s disease, which correlated with behavioral deficits and neuronal cell loss in the substantia nigra (Hsieh et al. 2015), therefore pointing toward a role of dopamine in rTMS-induced plasticity. On the other hand, several animal studies (in vitro and in vivo) indicate stimulus- and site-specific rTMS effects on the expression of neuromodulators, their receptors, and transporters (e.g., Ben-Shachar et al. 1999; Erhardt et al. 2004; Ikeda et al. 2005; Keck et al. 2002; Kole et al. 1999; Zangen and Hyodo 2002). A better understanding of the role of neuromodulation in rTMS-induced plasticity may thus support the development of novel means in early diagnosis, prognosis, and therapy of brain diseases, e.g., by combining pharmacological neuromodulation with specific rTMS protocols.
2.8 Translation into Clinics and Future Directions
As outlined in this book, numerous clinical studies have investigated and confirmed the therapeutic potential of rTMS in various brain diseases (see also Lefaucheur et al. 2014). However, our knowledge of the cellular and molecular mechanisms underlying rTMS-based therapies remains limited. Considering experimental advances in this field of research during the past decade, a biologically driven attempt to improve the use of rTMS in clinical practice has started to emerge, which may also help to better understand the considerable degree of inter- and intraindividual variability of rTMS effects seen in human subjects (see Chap. 1). However, this attempt can only go hand in hand with a better understanding of the role of neural plasticity under pathological conditions (Maggio and Vlachos 2014). For example, it remains unclear through the induction/modulation of which form(s) of plasticity (i.e., Hebbian plasticity, homeostatic plasticity, metaplasticity) rTMS could assert its beneficial effects in the course of a neurological or psychiatric disease (Müller-Dahlhaus and Vlachos 2013). In this context, rTMS effects on non-neuronal cell types need to be considered as well. To successfully transfer knowledge on the cellular and molecular mechanisms of repetitive magnetic stimulation into more effective therapies in neurological and psychiatric patients, it will be also important to study rTMS effects in animal models of brain diseases (e.g., by using genetic mouse and rat models of depression; Barkus 2013). We are confident that these studies will help building evidence-based frameworks for the clinical use of rTMS in the future (for review, see Nitsche et al. 2012).
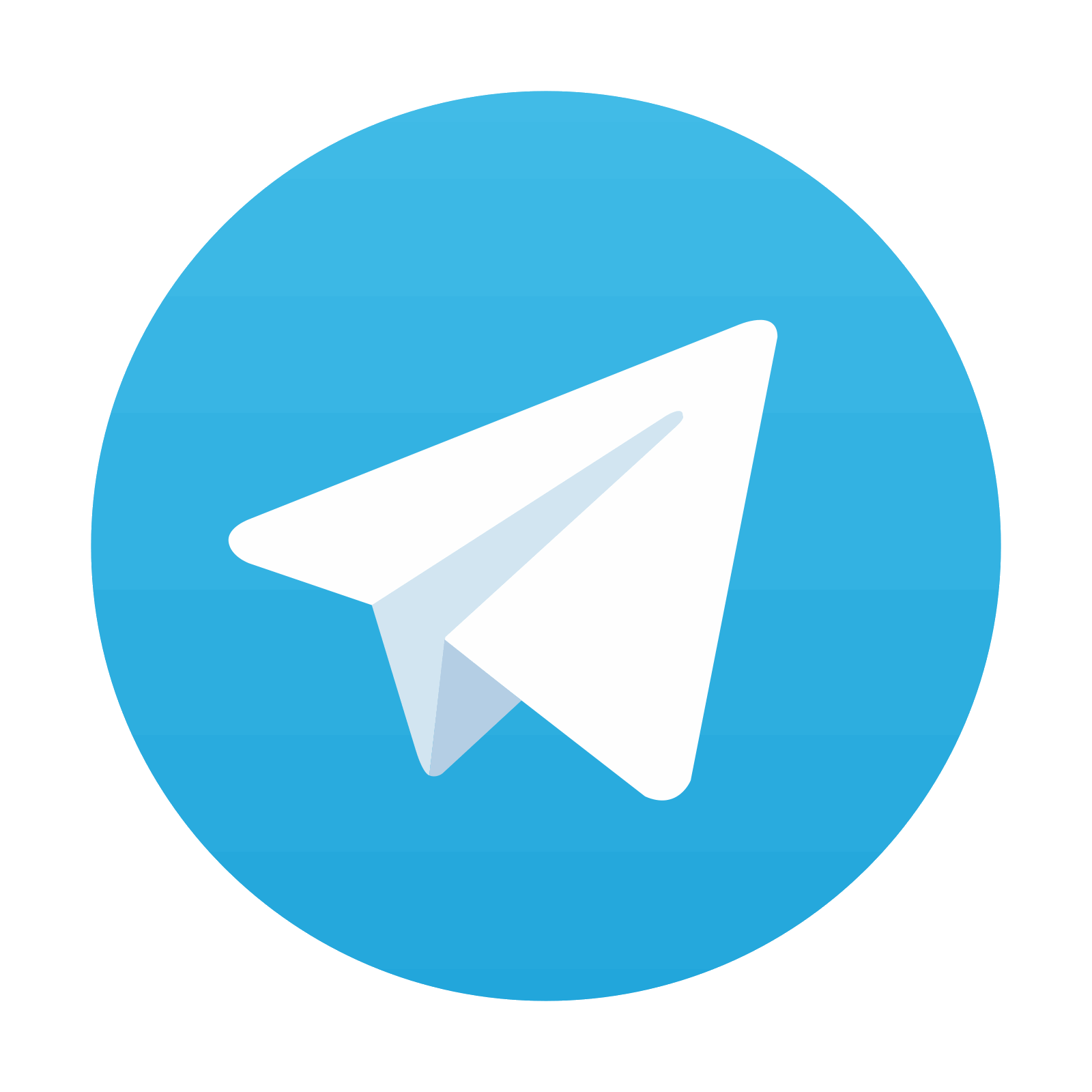
Stay updated, free articles. Join our Telegram channel
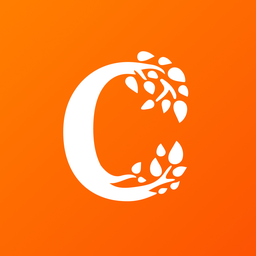
Full access? Get Clinical Tree
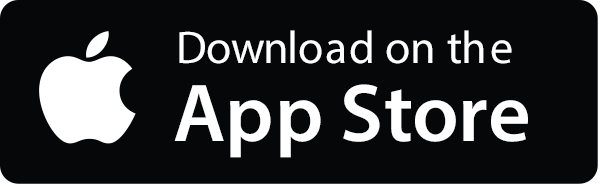
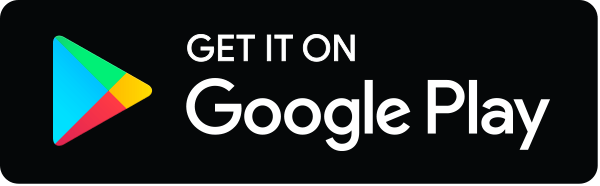