Abstract
Brain functions strictly depend on metabolites that cannot be stored inside the brain itself. Therefore, brain perfusion is regulated to keep the flow constant according to the brain activity and within a wide range of conditions. This autoregulation may be impaired following brain injuries or strokes, so monitoring the cerebral blood flow is crucial to avoid hypoperfusion leading to ischemia. This chapter will review the several different available methods for assessing cerebral blood flow, trying to enlighten strengths and drawbacks for each monitoring.
Keywords
Brain perfusion, Cerebral blood flow, Hypoperfusion, Kety–Shmidt, Xenon133
Contents
Introduction 32
Cerebral Metabolic Parameters 32
Autoregulation 33
Importance of CBF Monitoring 35
CBF Monitoring 35
Indirect Measurement Techniques 36
Neurological Examination 36
Neurophysiologic Monitoring 36
Transcranial Doppler 37
Laser Doppler Flowmetry 38
Thermal Diffusion Flowmetry 39
Near-Infrared Spectroscopy 40
Jugular Venous Saturation 41
Brain Tissue Oxygen Monitoring 42
Direct Measurement Techniques 43
Neuroimaging-Based Direct Measurement Techniques 48
Computerized Tomography Perfusion 48
Xenon-Enhanced Computer Tomography 50
Positron Emission Tomography 50
Single Photon Emission Computed Tomography 51
Perfusion-Weighted MRI 52
Arterial Spin Labeling 52
Other MRI Techniques 54
Future Directions 54
References 54
Introduction
Brain perfusion is peculiar, reflecting the specificity of the brain in the human body. Brain metabolic function depends strictly on oxygen and glucose, yet it is not able to store them, meaning that all the energy must be supplied through blood flow, which must be tightly controlled to avoid hypoperfusion and ultimately ischemia.
Despite representing just 2% of the total body weight, the brain receives 15% of the cardiac output, resulting in a cerebral blood flow (CBF) of about 50 mL/100 g every minute. This is an average value between the flow received by the grey matter (70 mL/100 g every minute) and the flow received by the white matter (20 mL/100 g every minute). Such a discrepancy is due to the different metabolic activity of the two tissues. In fact, although glial cells make up almost half the weight of the brain, because of their low metabolic rate, their energy consumption is only 10% of the total energy used by the brain itself. On the other hand, neurons require a high amount of energy and nutrients to keep their membrane potential and to ensure the synaptic activity, explaining why the perfusion they receive is so high.
Cerebral Metabolic Parameters
As mentioned previously the brain has no significant storage capacity; therefore CBF is tightly coupled with cerebral metabolism and oxygen extraction. This may be expressed by the equation:
CMR O 2 = CBF × AVD O 2
- •
CMRO 2 is the cerebral metabolic rate for oxygen, an index of cerebral metabolism. In normal conditions, this value is 3.2 mL of O 2 /100 mL of blood
- •
AVDO 2 is the arteriovenous difference of oxygen. This parameter is an index of oxygen extraction and normally is about 6.5 mL of O 2 /100 mL of blood
To gain a better understanding of the problem, we can use a simple mathematic model to describe the regulation of the CBF. In this kind of model, CBF is determined by the relationship shown in Eq. (2.2) :
CBF = MAP − ICP CVR = CPP CVR
- •
MAP is the mean arterial blood pressure ( Fig. 2.1 ).
Figure 2.1
CBF pressure autoregulation.
From Peterson EC, Wang Z, Britz G, Regulation of cerebral blood flow. Int J Vasc Med 2011; 2011 :823525.
- •
ICP is the intracranial pressure, which has a great influence on the back pressure of the intracranial venous system because the brain sits in a closed cavity where the increase of pressure may cause the venous system to collapse.
- •
CPP is the cerebral perfusion pressure.
- •
CVR is the cerebrovascular resistance defined by the diameter and length of blood vessels and by the blood viscosity.
This simple formula shows how, through its determinants, CBF may be affected by many physiological or pathological conditions, both systemic and intracranial.
Autoregulation
The brain has a strong tendency to keep the AVDO 2 constant. To reach this target the cerebral circulation acts by increasing or reducing CVR in response to the modifications of MAP (pressure autoregulation), blood viscosity (viscosity autoregulation), and cerebral activity (flow metabolism coupling).
Pressure Autoregulation
The brain circulation is able to maintain constant the CBF within a wide range of variation of MAP by modifying the diameter of the cerebral vessels, increasing or decreasing CVR. As shown in Fig. 2.2 , CBF is guaranteed between 50 and 150 mm Hg. Outside these limits the CBF varies according to the variation of MAP. The precise mechanism of this regulation has not been explained yet and will not be discussed in this work.

Viscosity Autoregulation
Blood viscosity is determined by many factors including hematocrit, plasma viscosity, erythrocyte and platelet aggregation and erythrocyte deformability. Blood rheology affects the blood flow, and hematocrit is the main determinant.
Since blood viscosity is inversely proportional to CBF, a decrease in hematocrit from 25% to 35% leads to an increase of 30% in CBF as result of vasodilatation. This autoregulation is kept until a hematocrit of 19% and further hemodilution does not increase CBF for the exhaustion of this compensatory vasodilatation.
Flow Metabolism Coupling
Since the end of the last two centuries, it has been well known that CBF depends on brain activity and metabolism. The AVDO 2 tends to increase with higher brain activity, and this tendency is compensated by the reduction of CBR, leading to a higher CBF. Again the mechanism between these regulations is complex and not yet completely understood.
Other Factors
Many other factors participate in regulation of CBF:
- •
metabolic mediators
- •
chemical
- •
PaCO 2 : has a powerful vasoconstrictor effect on brain vessels. Within a physiological range, for each kPa increase of CO 2 in arterial blood, there is a reduction of 15 mL/100 g every minute in CBF
- •
PaO 2 : in physiological conditions PaO 2 does not affect CsBF. However, when PaO 2 falls below 50 mm Hg, there is a quick rise in the CBF growth
- •
- •
temperature: hyperthermia affects CBF, increasing it by 6%–7% for every 1°C. Hypothermia has the opposite effect
- •
neural control
- •
circulatory peptides
Importance of CBF Monitoring
Besides all the possible physiological changes in CBF, this parameter takes on a great importance in pathological conditions as well. For instance, patients with intracranial disease that causes an increase in intracranial pressure are particularly susceptible to a decrease in CBF, possibly leading to ischemia. In this case, monitoring may be crucial in avoiding secondary ischemic lesions.
The perfect CBF monitoring method should have great temporal resolution to quickly detect ischemia and induce a clinical intervention to avoid it. Furthermore, it should be noninvasive, cheap, repeatable, inexpensive, and portable.
Unfortunately, a technology including all these features is not available yet, and there is still a need to accept some compromise.
CBF Monitoring
Currently, available CBF monitoring techniques may be divided into direct techniques (CBF is directly measured) and indirect techniques (CBF is derived as a result of calculation from its determinants) ( Table 2.1 ).
Noninvasive or minimally invasive | Invasive | |
---|---|---|
Direct |
|
|
Indirect |
|
|
Indirect Measurement Techniques
Neurological Examination
A neurological clinical examination evaluating the onset of neurological alterations is the easiest and more commonly available technique to indirectly assess a reduction of CBF. However the clinical examination is not sensitive enough to detect the ischemic process in its early phases nor specific as an indicator of CBF impairment.
Neurophysiologic Monitoring
Electroencephalographic waveform (EEG) alterations have been correlated to a reduction of CBF both in ICU and surgical settings.
When normal CBF declines below 25–35 mL/100 g/min the EEG trace first loses the fast frequencies; then, there is an increase in the slow rhythm. Below 12–18 mL/100 g/min, where the ischemic threshold sits, the delta activity becomes prevalent, and for further reductions, when the ischemic brain damage may become irreversible, there is the onset of suppression phenomena ( Fig. 2.2 ).
Quantitative EEG techniques (qEEG), like bispectral index, have been also used for CBF monitoring. Despite the ease of use of this instrument and the possibility to set up auto alarms, which is really useful in an ICU context, the evidence for this kind of use is limited and, due to the position of electrodes on the forehead, only frontal structures can be investigated.
In conclusion, EEG is a powerful monitoring technique as it may be used bedside for a continuous evaluation of CBF. Continuous EEG, especially if paired with clinical examination, is sensible enough to reveal a dangerous reduction in the CBF before the onset of ischemic phenomena. Its use may be limited by the requirement of a high skill to detect the ischemic alteration in the trace. Another drawback, particularly in an ICU setting, is the possibility of artifacts from sedative medications, seizures, temperature changes, or interference with other monitoring systems.
Transcranial Doppler
Transcranial Doppler (TCD) is a simple, noninvasive, and bedside technique that can indirectly estimate the CBF. This technique was introduced in 1982 by Aaslid et al., as evolution of TCD was performed, until that moment, only in children through the open fontanels.
To obtain a better penetration through the bone of the skull a 2-mHz linear probe is used to perform this technique.
The TCD follows the same principles of the extracranial use of this instrument: the soundwaves emitted by the probe are reflected by the red blood cells moving inside the vessels, and this reflection is captured by the transducer. The obtained signal is proportional to the velocity of the blood. Unfortunately, because of the low penetration of the soundwaves, it is not possible to study the image through the duplex ultrasound (B-mode), so the diameter of the artery may not be examined. Consequently, the given values are not absolute flow parameters, but they are rather relative speed values calculated by the formula for the Doppler shift. So, TCD provides information about the speed of the blood flow inside the scanned artery, and this reflects the relative CBF. It is important to remark that this hypothesis is true only if the resistance inside the vessel and the insonation angle remains constant.
Normally the flow is investigated inside the middle cerebral artery through a transtemporal window. Indeed, the flow in this artery is less influenced by the changes in systolic blood pressure, PaCO 2 , vasoactive, or sedative medications ( Fig. 2.3 ). This helps reduce one of the principal confounding factors, which, as said before, is the diameter variation in the investigated vessel.

The strengths of TCD are its wide availability in the ICU units, portability, and the low cost of the technique; moreover the testing may be repeated as it is simple, and no radiation is delivered to the patient, making it possible to obtain a circa-continuous measurement. On the other hand, TCD suffers from poor spatial resolution due to the fact that only the larger basal arteries can be studied with this technique, so the values express an estimation of the global CBF. Another drawback is that the retrieved values are relative. Finally the choice of the acoustic window is crucial, and in up to 15% of the population, it is inaccessible; therefore TCD cannot be performed.
Laser Doppler Flowmetry
Laser Doppler flowmetry (LDF) is an invasive indirect technique that provides continuous and real-time monitoring of microcirculation. The technique had been first introduced in 1980 for skin microcirculation, and at the beginning of the 1990s, it was applied in neurological ICUs for CBF monitoring.
LDF is another Doppler principle–based technique. A flexible fiberoptic probe is inserted through a craniotomy or a burr-hole inside the skull ( Fig. 2.4 ). The probe delivers monochromatic laser light with a wavelength of 780 nm, and this light is scattered by the tissues. The light reflected by static tissues maintains the same wavelength, while the light scattered by the moving red blood cells incurs a frequency change due to the Doppler effect. The Doppler shift of frequencies is linearly correlated with the speed of blood flow. Thus, considering the hemoglobin as a constant value, we can assume that the speed matches the flow.

LDF is an attractive technique for the given possibility of a bedside, continuous, and real-time monitoring of flow at a cellular level. It is also not radioactivity-based and relatively inexpensive. However, many limitations impact the usefulness of LDF. The main drawback is the small volume of brain wherein the flow is assessed (about 1 mm 3 ), so measured flow is an expression of local microcirculation. This may lead to artifacts if the probe is inserted in ischemic areas or if the brain is affected by perfusion alterations. Thus, every assumption about global CBF should be very cautious. One more trouble is introduced by movement artifacts (from the patient or from the probe) that can derange the values. Finally the CBF is only assessed by qualitative means, and last but not least, the technique is invasive.
Thermal Diffusion Flowmetry
Thermal diffusion flowmetry (TDF) is an invasive technique that uses thermal clearance to provide an estimation of brain perfusion.
Carter et al., 1991, introduced the TDF for cerebral surface monitoring, and the technique has been later refined with intraparenchymal measurement.
The theory underneath TDF is thermal clearance from the blood. A probe made up of two metal plates is inserted inside the brain. The distal plate (active thermistor) is heated and measures the blood flow by analyzing the heat transfer to the capillaries. The proximal plate (passive thermistor) is a thermometer measuring the baseline temperature. The difference of temperature decreases, while the flow increases; thus, it is possible to assess the CBF by a simple calculation.
TDF is a simple and effective technique providing real-time and continuous assessment of CBF. The intraparenchymal probes benefit from a very high temporal resolution, although the spatial resolution is poor and the measurement is local (4–5 mm around the thermistors). The CBF values are relative, but the instrument can be calibrated against 133 Xe or Xe-CT to obtain absolute values ( Table 2.3 ).
The placement of the probe is a critical process as tissue damage, bleeding, or infections are potential complications derived from the use of TDF. Furthermore the positioning over a vessel or a hematoma, movements, and sudden temperature changes (e.g., for rapid fluids infusion) may generate artifacts in the flow analysis.
Near-Infrared Spectroscopy
Cerebral near-infrared spectroscopy (NIRS) is an optical, noninvasive method for indirect estimation of brain oxygenation and perfusion.
NIRS for cerebral monitoring was first described by Ferrari et al., in 1985, and the first commercial available devices were introduced about 10 years later.
This technique makes use of a laser, emitting light wave frequencies within 600 and 1000 nm to enlighten the brain tissue through the skin and the skull ( Fig. 2.5 ). The choice of this precise spectrum is done to get the maximum penetration inside the tissue. Hence the near-infrared light is transmitted several centimeters inside the brain, where it is partly absorbed by the chromophores (mainly hemoglobin) and partly scattered. Even if the scattering introduces an additional complexity, at the same time, it is also useful. In fact the brain is too large to permit transillumination, but since part of the light is scattered back to the surface, a detector placed 4–7 cm away from the emitter is able to receive this light. From this signal, by the modification of the Lambert–Beer law (to consider the scattering phenomenon), it is possible to calculate in real time the relative concentration of chromophores inside the analyzed tissue.
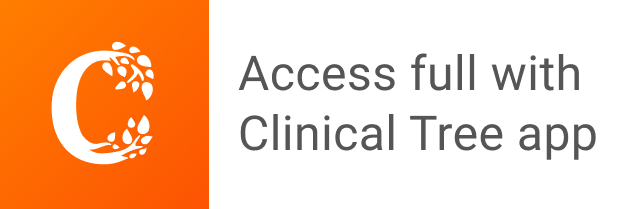