Summary of Key Points
- •
Cervical scoliosis is a rare condition that is a result of a structural spine deformity, often a congenital anomaly, neuromuscular pathology, neoplasia, traumatic injury, or prior spine surgery.
The vertebral or spinal column provides humans with the ability to maintain an upright posture, protects the neural and visceral organs (i.e., heart, lungs, abdominal contents), and aids with mobility. The cervical spine provides a transition from the rigid thoracic spine to the cranium and supplies the ability to alter position and thereby improve the swallowing function and optimize hearing and sight. Numerous forces, both internal and external, may affect the structure and position of the cervical spine such that it becomes deformed or is altered from its normal anatomic alignment.
Spinal deformities are more often encountered in the thoracic and lumbar spine. However, the cervical spine may also develop structural deformities secondary to congenital disorders, neuromuscular diseases, trauma, neoplastic disease, or previous spine surgery. These deformities may also occur in patients with systemic arthritides such as ankylosing spondylitis and rheumatoid arthritis. This chapter reviews the normal subaxial cervical anatomy, alignment, biomechanical properties, etiologies of scoliotic deformity, and treatment strategies.
Anatomy of the Cervical Spine
The cervical spine consists of seven vertebrae (C1-7) ( Fig. 148-1 ). C1 and C2 are anatomic and functionally unique, which allows for the transition and attachment of the cervical spine to the cranium. These vertebrae (C1-2) are not considered when discussing the subaxial cervical spine (C3-7).

Vertebral Body and Disc
The size of the subaxial cervical vertebral bodies (C3-7) generally increases from rostral to caudal (see Fig. 148-1 ). The exception is the C6 body, which is slightly decreased in size compared with C5. This increase in the vertebral body’s width, depth, and total end plate cross-sectional area allows greater loads to be supported and forces to be dispersed. The majority of these physiologic loads are carried through the ventral cervical vertebral body in a flexion posture, whereas the loads are carried through the dorsal elements (articular columns) in extension.
The intervertebral disc connects the vertebral end plates and is composed of the cartilaginous end plate, annulus fibrosus, and nucleus pulposus. The disc heights are asymmetric, with the ventral height being greater than the dorsal height (see Fig. 148-1 ). This contributes to the lordotic curvature of the cervical spine.
The pedicles are horizontal columns of bone that connect the vertebral body to the dorsal elements ( Fig. 148-2 ). The cervical pedicles have an elliptical shape, with the height being greater than the width. The cortical bone surface of the cervical pedicles is similar on the rostral and caudal portion, but the lateral wall thickness is significantly less than the medial wall thickness. These pedicles insert into the vertebral body with transverse angle ranges of −8 to +11 degrees from the horizontal and sagittal angles ranging from 40 to 29 degrees.

Articulations
There are four articular surfaces between adjacent vertebral segments in the subaxial cervical spine, one set located on the vertebral body and another set involving the dorsal elements. The articulations located on the rostral, lateral dorsal aspect of the vertebral body are termed the uncovertebral or Luschka joints. These “joints” articulate with the caudal, dorsal, lateral aspect of the rostral vertebral body ( Fig. 148-3 ). The heights of the uncinate process gradually increase as one descends the spine at each segment from C3-7, whereas the length and width remain relatively constant. These joints are actually believed to be degenerative clefts and not true joints because they are not present at birth and develop during adolescence.

The dorsal elements of the cervical spine allow a large degree of mobility due to a pair of segmental articulations in the form of facet joints (see Fig. 148-3 ). These are apophyseal joints and are composed of a loose but strong capsule and synovial lining. The cervical facet joints are oriented at approximately 45 degrees in the coronal plane and 80 to 90 degrees in the sagittal plane. This facet orientation permits a large degree of sagittal plane motion, flexion, and extension but limits or restricts translation and lateral movements or bending.
Ligaments
The anterior longitudinal ligament (ALL) and the posterior longitudinal ligament (PLL) are the two major ligaments in the cervical spine and are attached directly to the vertebral body. The ALL is a fibrous band that attaches to the edges of the vertebral bodies (C2 to sacrum) and is diminished in width at the disc spaces. It provides significant support and resists cervical extension. The PLL also is continuous from C2 to the sacrum but differs from the ALL in that it narrows over the vertebral bodies and then widens at the disc interspace where it is interwoven with the anulus fibrosus. This ligament resists flexion and has half the strength of the ALL.
Numerous other ligaments also support the dorsal cervical spine. These include the ligamentum flavum and the capsular and interspinous ligaments. The ligamentum flavum extends from the undersurface of the lamina to the adjacent rostral lamina. This ligament has the highest amount of elastin in the human body. It also has a baseline amount of strain in the neutral position such that with extension, the ligament does not relax and buckle into the spinal canal. The capsular ligaments attach circumferentially around the facet joints and are the strongest ligaments in the cervical spine. The interspinous ligament is relatively weak and spans between adjacent spinous processes.
Normal Cervical Alignment
The cervical spine permits head rotation, flexion, and extension to maintain the line of sight while also placing the cranium over the pelvis and supporting a balanced upright posture. Hardacker and colleagues demonstrated that a plumb line dropped from the tip of the odontoid process would fall ventral to the seventh cervical vertebra, hence sagittal balance ( Fig. 148-4 ).

It is difficult to define spine deformity based on clinical observation alone. Gross deformities may be determined through observation of the relationship of the tragus to the spinous process of C7 in the sagittal plane. However, these physical examination findings have been found to be inaccurate in most circumstances. In general, surface contour has not been shown to correlate with vertebral body location and position. Therefore, radiographs are essential to attempt to understand and document spinal alignment objectively.
At present, there is no accepted standard measurement algorithm for sagittal or coronal cervical spinal curvature. The most commonly used method is the Cobb angle technique that places parallel lines from the caudal and rostral aspects of the vertebral bodies and then measures the intersection angle of these perpendicular lines ( Fig. 148-5A ). Inaccuracy of the Cobb method has been reported because it is based on the noncuboidal shape of the vertebral bodies, where the vertebral body end plate-to-dorsal cortical angle is greater than 90 degrees. Harrison used tangential lines to the dorsal vertebral bodies ( Fig. 148-5B ) and calculated the “normal” cervical (C2-7) lordosis to be 26 degrees (C2 and C7). Based on these results and engineering principles, he concluded that the tangential technique is more accurate at assessing the cervical angle than the Cobb method, which from C1-7, overestimated, and from C2-7, underestimated the lordosis.

Cervical Alignment : Neutral
The length of the cervical spinal canal measured in the sagittal plane during flexion (kyphotic posture) is greater than during extension (lordotic posture). Therefore, the normal cervical lordosis allows the neural elements to traverse the spinal canal through a shorter course without ventral compression. The lordotic curvature may also protect against neural injury because axial loads are dispersed dorsally onto the facet joints and large articular pillars, rather than the vertebral body (as seen in kyphosis).
A number of disease processes affect the spine, in particular the thoracic and lumbar spine’s sagittal balance, which, in turn, affects the entire spine’s sagittal balance. The flexibility of the cervical spine allows it the ability to compensate for misalignment of the thoracic and lumbar spine. Therefore, an increased lordotic cervical posture has been observed when there was a concurrent exaggerated thoracic kyphosis. This compensation permits the maintenance of the overall sagittal balance (i.e., head over the pelvis).
Hardacker and coworkers measured the cervical curvature of 100 volunteers and recorded the mean total cervical lordosis alignment (foramen magnum to C7 inferior end plate) as 40 degrees (standard deviation of 9.7 degrees) where the majority of the lordotic curve was at the C1-2 junction and only 6 degrees was present from C4 to C7. Despite no patient having an overall cervical kyphotic posture, 39% had a segmental kyphotic angle greater than 5 degrees, typically at C4-5 and C5-6, based on individual segmental angles analysis. Gore and colleagues also measured mean lordotic angles in the cervical spine of osteoarthritis patients from perpendicular lines of the C2 and C7 bodies and observed a 16- to 22-degree lordosis for men and a 15- to 25-degree lordosis in women. Others have measured the C2-7 lordotic curvature to be approximately 14 degrees. Although numerous studies have shown a wide range for cervical lordosis, slight head extension (0 to 13.9 degrees) has been shown not to affect the cervical spine alignment.
Overall, there is not an accepted range defined as “normal” for cervical posture. Although definitive angles have not been calculated, studies have shown that due to aging and degenerative changes, the cervical spine has an increase in the lordotic angle. This lordotic angle increases with aging, from approximately 15 degrees in the third decade to 22 to 25 degrees in the seventh decade.
Cervical Alignment : Dynamic Movement
The cervical spine, also, allows a great degree of flexibility, and dynamic images (flexion and extension radiographs) permit an assessment of the intersegmental motion related to this flexibility. Flexion and extension radiographs of normal individuals have shown that the greatest motion occurs at C4-5 and C5-6 and the least at C2-3. Lin and associates further demonstrated that the spine moved from a lordotic position in extension to a nearly parallel position with flexion, such that all intervertebral differences in angular displacement were less than 7 degrees and translation was less than 0.6 mm. The total range of motion (ROM) from C2 to C7 was reported to be from 50 degrees to greater than 90 degrees with a normal gaussian distribution and a mean of 67 degrees. This ROM is affected by the stiffening of the spine, which occurs throughout the normal aging process.
Degenerative Changes
Cervical spondylotic myelopathy is a pathologic process that affects the aging spine. Gore and colleagues showed that 90% of asymptomatic males, age 60 to 65 years, had degenerative changes on cervical roentgenographic studies. As a result of aging, the vertebral discs dehydrate, and the vertebral column loses height ( Fig. 148-6 ). This loss of height results in a decreased tension of the ligamentum flavum. The loss of tension on the ligament causes it to shorten and results in buckling of the ligament into the spinal canal, with possible spinal cord compression. Also, degenerative and osteophytic changes take place in the facet joints, along with the vertebral disc space junction. The result is a stiffening or rigidity of the cervical spine and a decreased ROM. Holmes illustrated this by showing that the spine segments with the greatest motion migrate from C5-6 to C4-5 with aging.

The overall alignment of the cervical spine should not assume a kyphotic posture due to the normal aging process (see Fig. 148-6 ). Gore and colleagues showed that, despite the decrease in the intervertebral distance, the overall lordotic curve (C2-7) increased with aging in 200 asymptomatic adults.
Biomechanical Principles of the Cervical Spine
The position, posture, and motion of the cervical spine are complex processes that direct and manipulate forces on and around the spine. These forces allow the cervical spine to maintain an upright and lordotic posture. Due to the significant mobility of the cervical spine, the displacement of loads can be variable through different anatomic structures depending on the position of the spine during loading. For example, applied axial loads, with the spine in extension, are dispersed through the facet and dorsal articular column, whereas in flexion the vertebral body supports the majority of the load.
The point or position in the vertebral body that all other points rotate about when a movement occurs is termed the instantaneous axis of rotation (IAR). This is not a static point but rather is dynamic and changes with position, posture, and direction of movements. Also, each segment has a unique IAR for every movement; it is influenced by spine alignment, anatomy, muscle, and loads exerted. White and Panjabi theorized that in the sagittal plane for flexion and extension, the IAR is located in the ventral portion of the vertebral body. Therefore, each vertebral body has its own IAR for each directional movement. The summation of all the IAR movements dictates spinal column orientation and motion ( Fig. 148-7 ).

These forces that act on the spinal column have both a direction and a magnitude and are therefore referred to as force vectors. The perpendicular distance between a force vector and the IAR is defined as the lever or moment arm. The combination of the force vector and the lever arm results in a bending moment about the IAR. Therefore, the IAR can be thought of as a fulcrum, such that with flexion all points ventral to it come together and all points dorsal spread apart (see Fig. 148-7 ).
The muscle and ligament complexes of the cervical spine have a significant effect on the support, motion, and stability of the cervical spine. The effectiveness of each ligament is not only related to the strength of that ligament but also to the moment arm through which the ligament acts ( Fig. 148-8 ). A weaker ligament with a longer level arm might provide more support and strength to the spine than a very strong ligament located on the spinal column, with a short lever arm.

Multiple studies have illustrated the importance of intact and functioning cervical musculature to support and maintain the cervical lordosis. Nolan and Sherk performed a biomechanical analysis of the extensor musculature of the cervical spine and demonstrated that the semispinalis muscle acted as a dynamic stabilizer and the removal of its attachments resulted in the loss of cervical lordosis. Iizuka and associates reattached the semispinalis cervicis muscle after laminoplasty and found that attachment of the extensor musculature on serial magnetic resonance images correlated with a maintained cervical lordosis in the postoperative period.
The majority of the cervical spine’s ligamentous and muscle complexes support and attach to the dorsal aspect of the spine. These structures, along with the laminae, provide a lever arm, which allows the cervical spine to maintain a lordotic curve. Panjabi showed that without muscular support, the osteoligamentous cervical spine would buckle and fail at only one fifth the weight of the human head. These cervical spine specimens (C0-T1) failed with increasing loads of only 11 N, whereas in vivo load testing has shown load ranges from 53 to 1175 N. These data have to be taken in the context that in vitro models comparing segmental vertebral body motions do not correlate well with in vivo data.
The human body’s center of gravity is located approximately 4 cm ventral to the sacrum. Therefore, in the sagittal plane, this center of gravity is ventral to the vertebral body. In the standing individual, a plumb line dropped from the tip of the odontoid process falls slightly ventral (0 to 2 cm) to the ventral surface of the C7 vertebral body (see Fig. 148-4 ). The cervical IAR is also located ventral to the vertebral bodies. Therefore, there is a constant attraction or force drawing or pulling the spine toward the center of gravity. Newton’s first law states that an object at rest tends to stay at rest while an object in motion tends to stay in motion with the same speed and in the same direction unless acted on by an unbalanced force. Therefore, as long as the spine has the ability to resist against these gravitational forces through a strong tension band in the form of the dorsal musculoligamentous complex, the spine will maintain a lordotic curvature. Otherwise, the cervical spine will gravitate to its lowest energy state or a kyphotic posture, which implies that the cervical spine, without resisting forces, would prefer to be located at the center of gravity or zero energy state.
The strong muscles and ligaments of the cervical spine are able to maintain this lordotic posture against the gravitational forces due to their increased distance or greater lever arm relative to the IAR (see Fig. 148-8 ). This increased distance creates a mechanical advantage that the muscles and ligaments use to maintain a lordotic curvature. If the muscles and ligaments are weakened due to either congenital diseases or iatrogenic causes (postsurgical), such a dorsal “anchor” has a less substantial effect. Then, despite the mechanical advantage provided by the increased lever arm, the cervical spine will migrate further ventrally and the result will be a loss of the lordotic curvature. The ventral migration toward the IAR causes the lever arm that the compromised dorsal muscles and ligaments are acting through to be shorter ( Fig. 148-9 ). The shorter lever arm and the weakened dorsal tension band, ligaments, and muscles fail to provide ample support. This, in turn, results in further progression of the kyphotic deformity.

This cycle of impaired muscles and shorter lever arm continues until a significant deformity with possible neurologic findings results. This sequence may be illustrated in individuals with Marfan syndrome. In this disorder, the affected patients have an increased laxity of their ligamentous structures and therefore are at an increased risk for abnormal cervical alignment. Hobbs and coworkers confirmed this result by showing that 36% of patients with this disorder had absence of the normal cervical lordosis.
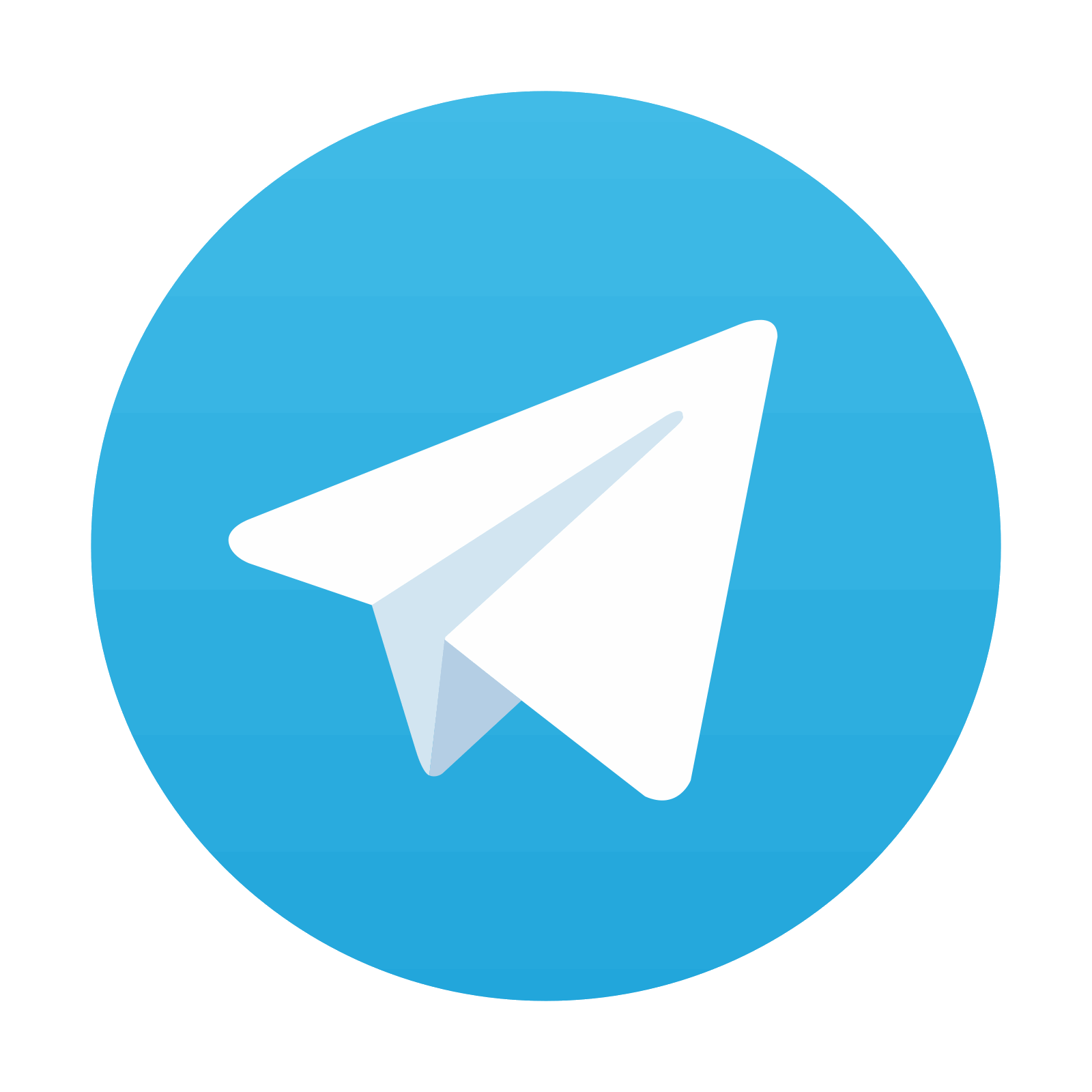
Stay updated, free articles. Join our Telegram channel
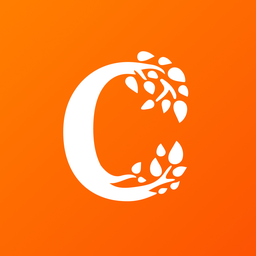
Full access? Get Clinical Tree
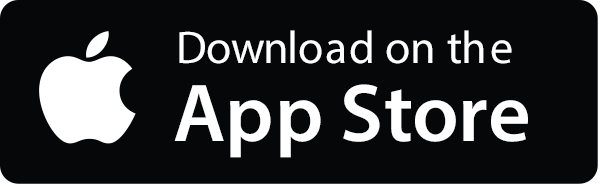
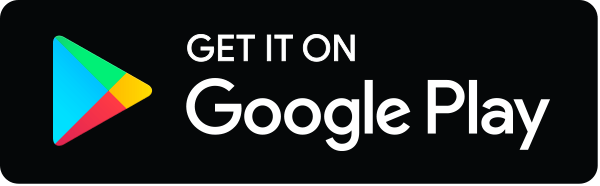