Abstract
Traumatic brain injury (TBI) is a leading type of epilepsy with significant repercussions for the quality of life of patients, due to the associated injury, consequent epilepsy and cognitive, behavioral or neuropsychiatric sequelae. There have been intense efforts to generate better strategies and methods to treat these patients better. This chapter reviews the advances in animal models of posttraumatic epilepsy (PTE), focusing on rodents, presenting an update on models, their phenotype, findings on neurobiology of TBI and PTE and future directions. The value of models, like the fluid percussion injury, controlled cortical impact, blast, penetrating TBI, weight drop TBI, in this process in being discussed as well as efforts to accelerate progress in the field through the use of collaborative research and infrastructure.
Introduction
Traumatic brain injury (TBI) is a common cause of emergency room visits for both adults and the pediatric populations, accounting for almost 2.5 million visits in the United States, a third of which are children.1 TBI causes diverse brain pathological changes, whether direct or secondary to the initial injury, which include cell death, axonal and mitochondrial injury, inflammation, gliosis, neurodegeneration and synaptic reorganization that have been associated with epileptogenesis.2–6 Seizures may occur immediately after the TBI (immediate or acute seizures) or during the first post-TBI week (early seizures). However, it is the occurrence of late spontaneous seizures, appearing after the first post-TBI week that establishes the diagnosis of PTE.7–10 Post-traumatic epilepsy (PTE) may develop in 2–50% of patients that experience TBI. The rate of PTE increases with severity of TBI and with longer observation periods.11, 12 Skull fracture, parenchymal brain lesions and intracranial hemorrhage, long period of post-TBI loss of consciousness, prolonged amnesia, age at TBI and – in several studies – acute post-TBI seizures have been linked with the development of PTE.13–16 In addition to PTE, TBI can be associated with cognitive, neurologic or psychiatric comorbidities, like depression or anxiety that also greatly impact the quality of life and medical care needs.17–23 PTE affects the patient’s quality of life due to epileptic seizures, deficits in memory and cognition,24–26 sleep disorders,27 post-traumatic stress disorder, depression, anxiety and social behavioral changes.28, 29 PTE is a devastating disease that still today remains without prevention or cure.6 The latent period for the development of epilepsy following TBI seems to be important as a time window for prophylaxis pharmacotherapies to prevent the development of epilepsy.6, 30, 31 The validation of methods that identify the time window for treatment and efficacy of drug effects is essential to translate to clinical trials. In this regard, studies with animal models are critical to elucidate the underlying mechanisms and pharmacological targets for therapeutic interventions in controlled experiments prior to clinical trial with epilepsy patients.
Here, we will review different animal models for TBI, with special emphasis on those that describe PTE development in rodents, highlighting the importance of each of them in the biological aspects post-trauma, comprehension of PTE development and preclinical trials for pharmacotherapies that may prevent and/or cure epilepsy following TBI.
A Comparison of Developmental Stages in Rodents and Humans
Rodents have a significant shorter life span, accelerated maturation and live in a different social environment than humans. The duration of gestation in rodents lasts 23 days opposed to 40 weeks in humans; eye opening occurs in postnatal days 13–15 (P13–15) rodents yet human newborns already have their eyes open; rodents start ambulating at 2 weeks whereas humans are able to do so at around 1 year of life; weaning from the dam in rodents takes place at P21 when breastfeeding in humans is usually during the first 6 months; life expectancy for rodents is up to 2 years whereas humans in the USA live approximately 80 years.32, 33 Comparisons of brain developmental stages in rodents and humans has produced different results, depending on the criteria used to stage these processes. Using the maturation of the hypothalamic–pituitary–gonadal axis as a criterion,32, 33 P0–6 are thought to correspond to the neonatal stage in rodents and P7–21 to infancy. Puberty begins around P32–36 in females and P35–45 in males, while adulthood is thought to begin after P60.34, 35 The observation that around P8–10 the rate of growth of the brain and its DNA, cholesterol and water contents in rodents resembles that of newborn human babies had led to considering the P-10 rodents as equivalent to human newborn babies.36, 37 However, discrete processes (timing of neurogenesis, synaptogenesis, gliogenesis, oligodendrocyte maturation, age-dependent behaviors, molecular and biochemical changes) mature with asynchronous trajectories within the brain across species challenging the concept that a single across-species staging method could apply for all developmental processes.32, 33, 38–41
Rodent Models of PTE
Fluid Percussion Injury
In the fluid percussion injury (FPI) model, a craniotomy is created at a specific skull region and when the animal starts recovering from anesthesia, it is connected to the fluid percussion device where a controlled pressure fluid pulse is delivered through the craniotomy, over an intact dura to generate TBI. Optimization and standardization of the conditions (anesthesia, size and location of craniotomy, pulse pressure) aim to simulate TBI of various severity levels and target specific brain regions. Acute mortality and acute post-TBI responses (apnea duration, first pain response, righting reflex), neurological exam outcome and acute post-TBI seizures are often used to describe the model characteristics. The FPI model reproduces several aspects observed in patients that suffered closed-head TBI with focal-diffuse injury.17, 42 Table 1.1 summarizes some of those findings in animal models.
Model | Sex, species, age | Induction method (anesthesia, method) | Gross pathology | Acute post-TBI seizure-like events and mortality | PTE rate and characteristics | References |
---|---|---|---|---|---|---|
Fluid percussion injury models: lateral (LFPI), rostral parasagittal (rpFPI), central (cFPI) | ||||||
cFPI | Male – SDR, adult | Day 1: Pentobarbital, midline 4.8 mm. Day 2: Methoxyflurane, FPI 2.1–3.8 atm |
|
| ND | 144 |
LFPI | Male – Harlan SDR, adult | Pentobarbital, LFPI 4 mm, severe (2.6–3.3 atm) | 30% of rats have acute post-TBI seizure-like events |
| 59, 63 | |
LFPI | Male – LE Hooded rats, adult | Isoflurane, LFPI 5 mm, moderate, severe, (3.2–3.5 atm) |
|
| 21, 51 | |
LFPI | Male – C57BL/6 mice, adult | Day 1: Pentobarbital, lateral parietal craniotomy 3 mm Day 2: Isoflurane, LFPI 2.9 ± 1.1 atm |
|
| At 6 months post-TBI
PTZ test, 7 months post-TBI
| 53 |
rpFPI | Male – SDR, P33–35 | Halothane, rpFPI 3 mm, 3.25–3.5 atm |
| 11% acute mortality | Grade 1–3 events:
‘Idiopathic seizures’,
| 61, 145 |
Controlled cortical impact model | ||||||
CCI | Male – SDR, adult | Isoflurane, CCI right parietal, 4 m/sec, 100 msec, 2.5 mm depth |
|
| Monitoring done for 356–404 hr Video monitoring:
Video-EEG monitoring:
| 71 |
CCI | Male – C57BL/6 mice, adult | Pentobarbital, left lateral parietotemporal craniotomy 5 mm. CCI done 45 min later (3 mm tip, 0.5 mm depth, 5 m/sec, dwell time 100 msec) |
|
| At 6 months post-TBI
Mean SRS duration 50±14 sec
PTZ test, 7 months post-TBI
| 53 |
CCI | Male – CD-1 mice, adult | Isoflurane, left lateral craniotomy 5 mm, CCI 3 mm impact tip, 2 mm depth, 5 m/sec, 100 msec |
|
|
| 69 |
CCI | Male – Harlan CD-1 mice, adult | Isoflurane, lateral craniotomy 4 mm, CCI 3 mm tip, 0.5–1 mm depth, 3.5 m/sec, 400 msec duration |
| Video monitoring only, starting 42 days after CCI
| 70 | |
CCI | Male – C57BL/6 J mice, P21 | 2,2,2-tribromoethanol ip or isoflurane, CCI Severe: 4.5 m/sec, 1.73 mm depth, 150 msec Moderate: 4 m/sec, 1.2 mm depth, 150 msec |
|
| 80 | |
Other models | ||||||
Blast TBI | Male – C57BL/6 mice, adult | Ketamine, dexmedetomidine, blast injury, 14.6 psi, Repeated TBI 3× | Increased Iba1, tau and tau phosphorylation in the brain |
| Intermittent EEG monitoring for months post-TBI
| 90 |
Penetrating TBI | Male – Charles River SDR, adult | Isoflurane, Steel burr 1.5 mm diameter 1000 revolutions/min, pushed in brain 8 mm below surface; copper or stainless steel wire or nothing placed in lesion | Lesion across tract (2 mm). Rats with seizures had more extensive lesion to ventral areas, amygdala and piriform Copper wire resulted in larger lesions and discoloration with copper precipitation | Early seizures, days 3–5 post-TBI (nonconvulsive):
|
| 94 |
CCI: controlled cortical impact; cFPI: central fluid percussion injury; FPI: fluid percussion injury; LE: Long Evans; LFPI: lateral FPI; PTE: posttraumatic epilepsy; PTZ: pentylenetetrazol; rpFPI: rostral parasagittal FPI; SDR: Sprague Dawley rat; TBI: traumatic brain injury.
Studies that have utilized the FPI model for young rodents at different ages (PN17 and PN28) reported that the youngest animals appeared to have longer apnea period, higher mortality rate and hemodynamic changes than adult rodents.41, 43 At older ages, the FPI is characterized by substantial structural damage to the impacted cortex (left parietal or right frontoparietal) and subcortical structures.6 The FPI model has also been applied to neonate (PN1–5) and juvenile (3–4 weeks old) pigs for the investigation of the different cerebral hemodynamic responses after TBI.41, 44
Several behavioral comorbidities are observed in FPI model that are similar to what is experienced in TBI patients (Table 1.2). In both rats and mice of both sexes, FPI results in cognitive deficits,18, 19, 21, 45–52 sensorimotor deficits,18, 50, 53–55 sleep abnormalities,56 anxiety18, 21, 50, 52, 53, 57 or depressive symptomatology.18, 19, 50, 57
Table 1.2 Models of TBI with comorbidities
Model | Sex, species, age at TBI | Cognitive, behavioral comorbidities | References |
---|---|---|---|
LFPI | Rats, adult |
| 21, 51 |
LFPI | Rats, P17–19 | Cognitive deficits Depression-like behavior Anxiety-like behavior Sleep abnormalities Locomotor impairment | 43, 48, 146–148 |
cFPI | Male – SDR, adult | Locomotor deficits 4–8 days post-TBI | 144 |
CCI | Male – SDR rats / adult | Spatial learning and memory deficits (MWM) Motor deficits | 149 |
CCI | Female – C57BL/6 mice, adult | Persisting impairment in spatial learning (MWM) Transient motor deficits (elevated narrow beam) | 150 |
CCI | Male – SDR rats, P28 | Spatial memory deficits (MWM) Short term memory (novel object recognition) Increased impulsive-like and anti-anxiety behavior (elevated plus maze) | 151 |
CCI | Male – SDR rats, P7 vs. P17 | P7: MWM deficits on day 11 post-TBI with more severe lesions P17: MWM deficits on day 11 post-TBI with all injury severities | 109 |
CCI | Male – C57BL/6 J Mice / P21 | Hyperactivity. Spatial memory deficits Social dysfunction | 80, 152 |
CCI | Male C57BL/6 mice, P21–25 vs. adult | Spatial learning deficits seen only in adult but not P21–25 injured mice (Barnes maze). No deficits in open field, light/dark test, tail suspension test found | 153 |
Closed head, weight drop, impact acceleration model | SDR rats, P17 | Visuospatial learning impairment (MWM) Locomotor deficits (beam balance, incline plane) | 105 |
Weight drop model, closed skull | Both sexes, SDR rats, P30 | Motor / balance impairment (beam walking, open field activity) – 1–2 days post-TBI Executive functioning deficits (novel context mismatch) – 5–8 days post-TBI Depression-like deficits (forced swim test) – 15 days post-TBI Decreased anxiety in males (elevated plus maze) – 1 day post-TBI Spatial learning (MWM) – 10–13 days post-TBI | 116 |
Cellular and molecular post-FPI changes that have been investigated for their role in epileptogenesis include alterations in the immune system, such as increased cytokine interferon gamma (IFN-γ),54 microglial activation and reactive astrocytosis,52 axonal injury at white matter tracts such as the corpus callosum,52 and decrease in protein phosphatase 2 A (PP2A) activity21 associated with overexpression of phosphorylated tau protein.21, 57, 58
The FPI model has been widely used as a model for PTE. The incidence of epilepsy after FPI depends on the severity, location of the injury, duration and time points used for monitoring for seizures, type of seizure-like patterns detected and the age of the animals. In studies from adult rodents that looked at EEG seizure rhythmic patterns with evolution in patterns, frequency, location, amplitude, the rate of PTE was more modest and increased with time from injury. In a longitudinal study utilizing video-EEG intermittent recordings in lateral FPI (LFPI) rats, the PTE rate was ~11% at 15–17 weeks post-injury, 30% at 7 months and ~50% at 12 months post-TBI,59 and these seizures also included more classical motor correlates. For example, in adult male LFPI rats,60 50% of the animals presented focal seizures, 17% had generalized seizures and 87.5% developed late focal seizures. Similar rates ranging between 30% and 50% at 6–12 months post-TBI were also described by other groups modelling severe LFPI injury.21, 51, 58, 60 In mice, the rate of PTE may vary according to strain. In C57BL/6S mice, 3% developed PTE till 9 months post-injury, whereas the majority (71%) developed interictal epileptic activity.41, 44, 53 In D’Ambrosio’s studies, using the rostral parasagittal FPI model in postnatal day 33–35 (P33–35, peripubertal) old rats, the reported seizure patterns consisted of focal or bilateral rhythmic activity occasionally with rhythmic spikes were described as grade 1 (focal, ipsilateral to lesion, brief), grade 2 (focal to bilateral) or grade 3 (bilateral) in up to 100% of the FPI animals by 9 weeks post-FPI.61 Behaviorally, these patterns were associated with no or mild manifestations (freezing, facial automatisms, head nodding or myoclonus). EEG patterns described as ‘idiopathic’ with rhythmic spikes at the parieto-occipital regions bilaterally were also seen in older animals, 27–28 weeks post-TBI and also in 33% of controls. Table 1.1 presents a summary of studies on PTE models.
A point of caution in such studies is the detection of bursts of spike-wave discharges in the various rodent cohorts associated with freezing, immobility and often terminated with sudden movement. These may resemble the absence-type seizure patterns seen in absence epilepsy models, can be focal or bilateral and can be seen also in cohorts of experimental controls, increasing with age.62 In an injured brain, such patterns may be altered in morphology or rate of appearance and it is highly recommended to have similarly monitored controls to establish the effect of injury on these naturally occurring patterns. Furthermore, rare occurrences of seizures in experimental controls undergoing video-EEG have been reported due to complications or predisposing conditions,62 emphasizing the need for similar video-EEG monitoring protocols in the study controls.
Although the FPI model is an important tool for the study of PTE development and screening of pharmacological interventions with potential to act in disease-modification and prevent the development of PTE, as all models, it has some limitations. To simulate the brain injury, a craniotomy is necessary to expose the brain. This procedure itself can be potentially the cause or part of the brain injury.54 Studies have shown that sham animals, exposed only to the craniotomy surgery, without the injury, had focal seizure after the procedure.60 In the same way, increase in cytokines, KC-GRO (growth-regulated oncogenes (GRO) / keratinocyte chemoattractant (KC)) and IFN-γ have been reported in sham animals compared to naïve.54, 59, 63 Optimization of the conditions for the induction of FPI injury is needed in each laboratory to ensure that the acute outcomes are equivalent to parameters used for the desired injury severity. This model has a high incidence of acute mortality post-injury, about 30% when severe, requiring a high number of animals to achieve power for statistical analyses.54, 59, 63
Controlled Cortical Impact (CCI) Model
The controlled cortical impact (CCI)64 model was developed in the 1980s to simulate human closed-head TBI in the ferret and create a test to determine changes in brain tissue after a direct mechanical deformation,65, 66 and it is widely used especially in rodents, generating cortical and hippocampal focal lesions.53, 54, 67–73 The CCI consists in impacting the cortical area through a stroke-constrained pneumatic impactor. This can be done either through a pre-existing craniotomy on an intact dura,65 or by impacting the intact skull.66 The tip of the impactor can vary in size and geometry, and CCI devices allow control of the speed, depth and duration of impact.66 All those parameters can determine the severity of the trauma, underlying pathology and the subsequent rate of PTE; it is therefore important to standardize these conditions in each laboratory when establishing the model.
Similar to the FPI, the CCI model leads to memory and learning deficits,67–69, 72 dysfunction in the motor and sensory systems,54, 69 and also affects psychosocial-related behaviors69 (Table 1.2). In pediatric CCI studies, cognitive impairment and sensorimotor deficits along with anxiety-like behaviors even 2 months after injury are typical in P17 rats that have been subjected to CCI. Neuronal reorganization and alterations in white matter may also ensue.74, 75 Recently, P21 mice were exposed to CCI, which led to neuropathological characteristics consistent with PTE, like mossy fiber sprouting and neuroinflammation resulting in cortical atrophy and hippocampal sclerosis. These mice also exhibited behavioral abnormalities such as hyperactivity, social impairment and memory deficits.76–79 In addition to that there was a strong increase in vulnerability to provoked seizures in response to intraperitoneal (i.p.) pentylenetetrazole (PTZ, GABAA receptor antagonist) administration, as early as 2 weeks post-TBI and persisting until at least 6 months later. Furthermore, more than 87% of the CCI mice had at least one generalized motor seizure during a 7-day video EEG recording at 4–5 months post-injury.6, 80
The rate of PTE post-CCI varies across studies, ranging between 9% and 50% depending on the injury severity, location, strain and criteria for seizure detection53, 69, 71 (Table 1.1). As in the FPI model, the rate of interictal epileptic discharges is significantly higher, up to 82%.53, 69 The CCI model is widely used, has similarly high mortality as the LFPI model of severe TBI (around 30% in severe TBI),54 and there is no injury parameter standardization.66
Blast-induced Traumatic Brain Injury (bTBI) Model
An explosion causes a high speed blast wave. When this blast wave hits one’s body it is absorbed and propagated causing widespread damage, including to the brain.81 TBI caused by blast can be correlated to three different events: primary blast injury – result of the wave air dislocation; secondary blast injury – impact of fragments due to the explosion; tertiary blast injury – when one is thrown away by the blast.82 Some experiments with animal models are done in free-field blast, where real explosives can be used in large animals as pigs and sheep.17 For rodents, usually a long metal blast tube with explosives is used to generate the blast wave and the animal is positioned in a specific distance of the explosion.82 The closer the explosion focus is, the higher is the severity and mortality of the model.83
The bTBI is not as widely studied as the FPI or CCI and may carry more inherent variability in the injury, both within and outside the brain, which may complicate the study design. However, it is a more realistic model for military field-based TBI caused by explosions. Similar to the other TBI models, it may result in inflammatory responses in the brain, elevation in glial fibrillary acidic protein (GFAP) in the ventral hippocampus, prefrontal cortex and amygdala.84 Levels of IFN-γ and interleukin-6 (IL-6) were increased in amygdala and ventral hippocampus.84, 85 After bTBI, rats had increase in anxiety-like behaviour,84, 86–88 and elevated levels of serum corticosterone84 as well as depression-like behaviors and cognitive impairments.84, 86–89 Spontaneous seizures have been described as more frequent in models of repeated (3×) bTBI than in single bTBI90 (Table 1.1).
Penetrating Brain Injury Model
Penetrating brain injury is caused by an object that penetrates the brain, for example, a gun bullet. This is the injury type with the higher risk of PTE development, especially if pieces of bone or foreign fragments are retained inside the brain.17 Besides the fragments in the brain, the bullet also makes a cavity larger than its own size. In order to simulate the penetrating ballistic-like brain injury, some studies were done using a custom probe inserted in the animal’s brain. The probe is connected to an elastic balloon on the tip that is quickly inflated inside the brain making thus the cavity similar to a bullet’s penetration.91–93
Anatomical and physiological changes due to the probe penetration and inflammation in the rat’s brain are seen. Intracranial hemorrhage and lesion size have been correlated to the injury severity as shown by increase in hemispheric swelling due to rise in intracranial pressure.93 Penetrating brain injury also leads to impairment in sensorimotor skills and spatial learning deficits in rats.92, 93 Likewise, this model caused incidence of nonconvulsive seizures and periodic epileptiform discharges, and both were positively correlated with the severity of the injury.91
The limitation of using the probe is that it does not mimic the penetration of fragments of bone or pieces of the penetrating object. To overcome this, a new model was developed using a high speed drill, whereby a 1-mm diameter steel burr was pushed through the exposed skull into the brain, from the dorsal cortex to the ventral hippocampus, and the fragments were not removed.94 Interestingly, the injured animals that received copper wire in the lesion were more likely to develop epilepsy (96% vs. 15% of the steel injured rats).94 Copper is present in bullets, suggesting that it may be an important inducer of PTE development after gunshot wounds.94
Weight-drop Models
Different weight-drop models have been developed over the years in rats and mice and are induced by a guided weight that is released to impact the animal’s head. Feeney’s model was the first to be developed and requires a craniotomy to expose the dura mater and mainly provokes a focal injury.4 The animal is positioned under a cylinder that contains a weight and a footplate. The weight is released and strikes the footplate that goes over the exposed intact dura, generating the brain injury.95 This trauma caused cortical contusion and white matter hemorrhage which progresses to a necrotic cavity that can expand over the time.95–97
The Marmarou’s impact acceleration model does not require a craniotomy but only a surgical procedure to expose the skull. A metal disk is glued on the exposed skull midline, between bregma and lambda, and acts as a helmet avoiding bone fracture. The animal is placed with the disc attached on a foam platform and a brass weight is released inside a Plexiglas tube on the disk causing a diffuse axonal injury.98 This type of injury is usually seen after falls or car accidents.4 Motor and cognitive impairments are observed after trauma.99, 100 Anatomical diffuse damage can be seen in axonal and dendritic structures, as well as injury in microvasculature.101 The limitations of these models are that there is a high variability of severity and the mortality is relatively high in severe injury, and it is caused basically by respiratory depression.4
Shohami’s model also consists of a focal closed-head injury and does not require a craniotomy. In this model, an incision is made to expose the skull and then a weight is released to fall upon one side of the unprotected rodent’s skull.102 The trauma caused by the weight drop leads to motor function, memory deficits, cerebral edema, hippocampal neuronal death and breakdown of the blood–brain barrier (BBB) in the mouse brain.103, 104 In this model, the device is easy to operate, however, it is not highly reproducible.4
In the pediatric version of the weight drop model, a helmet is often utilized so as to prevent penetration and later skull fractures, which are very common in small and young animals after injury.95, 98 Severe injury in this model can also mimic the extended swelling and neurocognitive impairment that is quite often seen in brain-injured children.105–107 Specifically, injury of P17 animals can lead to vestibulomotor deficit up to 10 days post-TBI, as well as remarkable axonal injury and astrogliosis and deficits in the Morris Water Maze test of visuospatial learning and memory, 3 months after the trauma108, 109 (Table 1.2).
TBI-related Brain Pathologies, Age Dependence and Relevance to PTE
TBI causes multifaceted and multifocal pathologies in the brain that may progress or evolve with time, setting the stage for the development of resultant neurological, functional deficits or epileptogenesis. Immediate changes include the structural lesions caused by TBI: skull fracture, dura deficits, axonal injuries and cell loss, disruption of the integrity of extracellular matrix and neurovascular units resulting in BBB disruption, hemorrhages and edema. The acute post-traumatic seizures, when present, or systemic disorders, such as hypoxia or metabolic changes during the acute and subacute critical post-TBI periods, may further exacerbate these pathologies. The subsequent cellular or molecular changes include cell proliferation (including neurogenesis), gliosis, synaptic remodeling and inflammation that further modify the structural and functional disturbances that define the long-term TBI outcomes. Both clinical and preclinical studies have suggested that these effects may depend upon the severity and nature of the TBI but also may be further modified by biological factors, such as age or sex. A limitation for across age comparisons stems from the striking anatomical differences (skull and brain size, skull thickness and integrity) that challenge the comparisons of the strength and propagation routes of the applied pressure pulses or the equivalency of other TBI induction methods. Regardless, we will discuss here selected examples of TBI-related pathologies and their potential relevance to long-term TBI outcomes, including PTE. In addition, in Table 1.3 we summarize the preclinical trials testing new treatments to modify PTE rate and severity.
Treatment | Mechanism | Model | Effects on seizures | References |
---|---|---|---|---|
Atipemazole | α2 adrenergic antagonist | LFPI, adult rats | No effect on spontaneous seizures Reduces PTZ seizure susceptibility | 154 |
Ceftriaxone | Antibiotic, increases expression of glutamate transporter GLT-1 | LFPI, adult rats | Reduced cumulative seizure duration 12 weeks post-TBI | 155 |
Focal cooling | Focal cooling 0.5–2°C | rpFPI, P32–36 rats | Abolished ictal activity up to 10 weeks post-TBI Ictal events: rhythmic spike waves with freeze-like arrest | 156 |
Rapamycin | mTOR inhibition | CC1, adult CD1 mice | Reduced PTE rate at 4 months post-CCI (by video-EEG monitoring) | 69 |
Rapamycin | mTOR inhibition | CC1, adult CD1 mice | No effect on behavioral seizures (visual monitoring only) | 136 |
Sodium selenate | Protein phosphatase 2 A activator | LFPI, adult rats | Reduced seizure frequency during and after treatment | 58 |
Kineret | Interleukin 1 receptor antagonist | CCI, P21 C57BL/6 J mice | No effects on spontaneous seizures, reduced PTZ seizure susceptibility | 80 |
The table is modified version of Table 1 in Saletti et al. (2019)6 and reproduced with permission from Elsevier.
CCI: controlled cortical impact; LFPI: lateral FPI; P: postnatal day; PTE: posttraumatic epilepsy; PTZ: pentylenetetrazol; rpFPI: rostral parasagittal FPI; TBI: traumatic brain injury.
TBI can increase neurogenesis in specific brain regions, such as the subventricular zone of the lateral ventricles and the dentate gyrus.110 Using the LFPI model, both the number of newborn cells at the subgranular zone of dentate gyrus as well as their differentiation to neurons was greater in rats injured at P18 (juvenile) than in adulthood.111 Similarly, CCI induced a stronger proliferative response ipsilateral to the lesion, when injury was induced at P6, P11 or P17 compared to adulthood.112 Whether early increase in post-TBI neurogenesis favors recovery or promotes epileptogenesis has been debated. A recent study suggested that early neurogenesis in the LFPI model in P23–25 Wistar rats promotes excitability and increases seizure susceptibility to induced seizures post-TBI.113
The formation of new dendritic arbors, synapses and synaptic pruning, that is the selective retention of synapses that are functionally important and useful, are critical steps for the normal brain development. Injuries may damage axonal and synaptic connections or may create aberrant connections that may contribute to epileptogenesis or comorbidogenesis. In CCI-injured adult rats, a 60% loss of synapses in the CA1 sector of the hippocampus was observed at 2 days post-CCI and a gradual, but partial recovery was noted till 60 days post-CCI.114 However, this post-TBI partial recovery in synaptogenesis did not translate in to improved spatial learning. Further, a characteristic pathology of hippocampal epileptogenesis (aberrant mossy fiber sprouting at the inner molecular layer) was reported in all CCI-injured adult rats.71 Age-dependent vulnerability to injury has been reported in the CCI model, whereby the morphology and complexity of dendrites was not affected after CCI in P7 rats as opposed to P17 and P30 rats, in which region-specific changes were observed.115–117 Other studies recorded a higher rate of synaptic sprouting in P35 rats compared to adult rats118 as well as plastic reorganization of circuitry in the injured brain cortex and hippocampus.75 This circuitry reorganization has been correlated with gradual lesion extension and transient behavioral phenotypes, suggesting the implication of post-traumatic plasticity to dysfunctional neuronal networks leading to proneness to seizures.119
Inflammatory signaling pathways have attracted attention for their potential role for epileptogenesis under different settings, including TBI 6, 120, 121 (Table 1.1, Table 1.3). It has been proposed that the developing brain elicits more robust inflammatory response in response to TBI, greater disruption of BBB and is less capable in counterbalancing oxidative stress or detoxifying free iron after TBI induced bleeding.120 In the CCI model, P21 C57BL/6 mice showed more pronounced acute and more prolonged cortical infiltration by CD45+ leukocytes or GR−1+ granulocytes in the developing brain than in the adult brain. Cortical volume loss was more pronounced acutely in adults but showed progressive deterioration in the injured P21 mice.122 Increased levels of Interleukin 1β (IL-1β) levels were observed in P21 mice in the cortex, hippocampus and serum during the first days after TBI; treatment with IL-1 receptor antagonist (IL-1Ra) improved spatial memory and susceptibility to evoked seizures, although no significant effect was seen over spontaneous seizures80 (Table 1.3).
The brain is highly susceptible to oxidative damage because of its high rate of oxidative activity and the relatively low antioxidant capacity. Enzymes such as superoxide dismutase (SOD), catalase and glutathione peroxidase act against the production of reactive oxygen species (ROS). In mice, catalase, total SOD and total glutathione peroxidase enzyme activities increase from the embryonic to the early postnatal (P1) period and either decline (total SOD, total glutathione peroxidase activity) or gradually increase till P21.123 In rats, the activity of these enzymes also increases between the embryonic to early postnatal days, but the trajectory of these changes differs thereafter, with SOD and glutathione peroxidase activities increasing through adulthood and catalase activity decreasing between P10 and adulthood.124–126 These suggest age- and possibly species-specific responses to insults that produce oxidative stress, such as TBI.
Recently, research has been focused on the effect TBI can have on cerebral metabolism mainly in the developing brain. Cerebral metabolic abnormalities in infants and children after closed head injury have been linked to poor neurodevelopmental outcomes.127, 128 A decrease in cerebral glucose metabolism has been described in P17–21 rats after CCI.129–131 An age-dependent switch from ketones to glucose as the main fuel source of brain probably contributes to the flexibility of the developing brain to metabolic disruption. Pre-weanling and even peripubertal rodents (~P35) have a better capacity for ketone uptake and metabolism than the adult animals, which possibly adds to the better metabolic response and neurochemical balance in the immature brain after injury.132, 133 A ketogenic diet has been tried in adult LFPI-injured rats and increased the threshold for flurothyl induced seizures but has not been tested on spontaneous seizures.134
There are several pathologies and pathways that have been investigated for their role in PTE epileptogenesis and post-TBI sequelae. The mechanistic target of rapamycin (mTOR) pathway is a critical hub in many epileptogenic pathways and has shown disease modifying effects in several models of epilepsy.135 In the CCI model, rapamycin has reduced the rate of PTE in adult mice in a study using video-EEG recordings but did not show efficacy in a trial using only visual monitoring for seizures.69, 136 Earlier studies, in the closed head weight drop model, showed functional and behavioral improvement with rapamycin.137
Traumatic brain hemorrhage is a well-known risk factor for the development of PTE, both due to direct structural lesions but also due to iron toxicity and/or accumulation and production of free radicals.6 Ferric chloride is a known precipitant of focal epileptogenesis when injected in the sensorimotor cortex.138 An intracortical iron injection TBI model has been developed and more excitatory neurotransmitters were reported in the brain of rats with seizures compared to those that did not manifest seizures.139 Since TBI can also result from trauma that includes introduction of metals in the brain, the role of metals has been investigated. Copper, for example, has been proposed as more epileptogenic than stainless steel in a penetrating injury TBI model94 (Table 1.1).
The hyperphosphorylation of tau protein, an integral pathology in many neurodegenerative disorders, post-traumatic encephalopathy and some epilepsies, has attracted a lot of interest for its potential contribution to both post-TBI comorbidities and PTE.2 The first preclinical studies demonstrated promising effects of drugs that reduce hyperphosphorylated tau in the LFPI model, in regards to ameliorating spontaneous seizures and post-TBI pathologies.21, 58 Pathological hyperphosphorylation of tau has been demonstrated in several models of TBI and remains an interesting pathology for future studies.140
The Operation Brain Trauma Therapy (OBTT), a multicenter preclinical consortium for the screening for treatments and biomarkers has investigated several candidate treatments for their effect in various TBI models, on neurological, cognitive and histology outcomes, as well as on serum biomarkers, like GFAP (glial fibrillary astrocytic protein) and UCH-L1 (ubiquitin carboxy-terminal hydrolase-L1).141 Among the drugs with the best profile included levetiracetam, which improved cognitive function in the LFPI and CCI models with excellent tolerability.141 Levetiracetam is commonly given during the acute phase of severe TBI patients to prevent early seizures and consequent harmful complications, although there is no evidence of antiepileptogenic effect for these drugs, preclinical studies indicated some benefit, possibly attributed to the antiseizure effect and reduction of inflammation.
There is currently no evidence for validated antiepileptogenic treatment in TBI. Several treatments have been tested preclinically (Table 1.3) though validation through additional studies needs to be done. The Epilepsy Bioinformatics Study for Antiepileptogenic Therapy, EpiBioS4Rx, is an National Institute of Neurological Disorders and Stroke (NINDS) funded international preclinical and clinical multicenter without walls that aims to create a platform for the identification, validation and translation of new treatments to prevent PTE through the use of biomarkers and rigorous multicenter studies in the LFPI model.142, 143
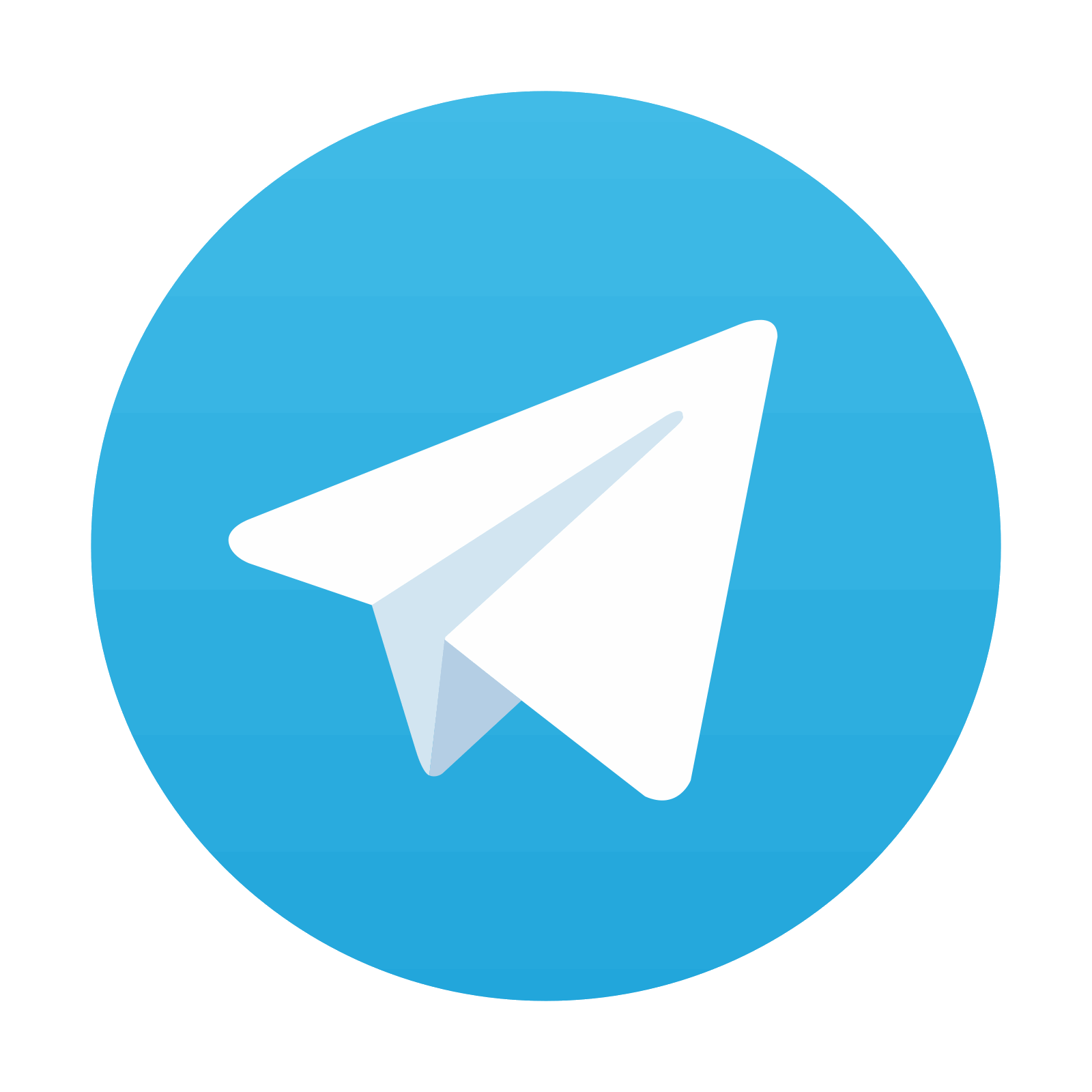
Stay updated, free articles. Join our Telegram channel
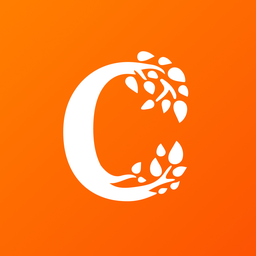
Full access? Get Clinical Tree
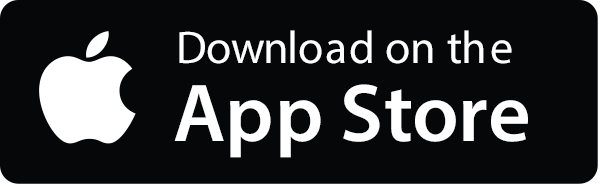
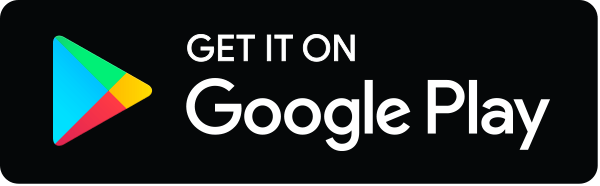