Neuropathology
Vascular Origin of Cerebrovascular Disease
In the latest edition of the International Classification of Diseases and Related Health Problems (ICD-11) cerebrovascular diseases (CVD) are listed in the section of diseases of the nervous system [1]. However, they have their origin in the vessels supplying or draining the brain, and the knowledge of pathological changes occurring in the vessels and in the blood are essential for understanding the pathophysiology and therapy of the various types of CVD. Changes in the vessel wall lead to obstruction of blood flow; by interacting with blood constituents they may cause thrombosis and blockade of blood flow in this vessel. In addition to vascular stenosis or occlusion at the site of vascular changes, disruption of blood supply and consecutive infarcts can also be produced by emboli arising from vascular lesions situated proximally to otherwise healthy branches located more distal in the arterial tree or from a source located in the heart. At the site of occlusion, opportunity exists for thrombus to develop in anterograde fashion throughout the length of the vessel, but this event seems to occur only rarely.
Changes in large arteries supplying the brain, including the aorta, are mainly caused by atherosclerosis. Middle-sized and intracerebral arteries can also be affected by acute or chronic vascular diseases of inflammatory origin due to subacute to chronic infections, e.g. tuberculosis and lues or due to collagen disorders, e.g. giant cell arteritis, granulomatous angiitis of the CNS, panarteritis nodosa, and even more rarely systemic lupus erythematosus, Takayasu’s arteritis, Wegener granulomatosis, rheumatoid arteritis, Sjögren’s syndrome, Sneddon and Behçet’s disease. In some diseases affecting the vessels of the brain, the etiology and pathogenesis are still unclear, e.g. Moyamoya disease and fibromuscular dysplasia, but these disorders are characterized by typical locations of the vascular changes. Some arteriopathies are hereditary, like CADASIL (cerebral autosomal dominant arteriopathy with subcortical infarcts and leukoencephalopathy), in some like cerebral amyloid angiopathy a degenerative cause is discussed. All these vascular disorders can cause obstruction, and lead to thrombosis and embolizations. Small vessels of the brain are affected by hyalinosis and fibrosis; this “small-vessel disease” can cause lacunes and, if widespread, is the substrate for vascular cognitive impairment and vascular dementia.
Atherosclerosis is the most widespread disorder leading to death and serious morbidity including stroke [2]. The basic pathologic lesion is the atheromatous plaque, the most commonly affected sites are the aorta, the coronary arteries, the carotid artery at its bifurcation, and the basilar artery. Arteriosclerosis, a more generic term describing hardening and thickening of the arteries, includes as additional types Mönkeberg’s sclerosis and is characterized by calcification in the tunica media and arteriolosclerosis with proliferative and hyaline changes affecting the arterioles. Atherosclerosis starts at young age, lesions accumulate and grow throughout life and become symptomatic and clinically evident when end organs are affected [3].
Atherosclerosis: atheromatous plaques, most commonly in the aorta, the coronary arteries, the bifurcation of the carotid artery and the basilar artery.
The initial lesion of atherosclerosis has been attributed to “fatty streaks” and the “intimal cell mass.” Those changes already occur in childhood and adolescence and do not necessarily correspond to the future sites of atherosclerotic plaques. Fatty streaks are focal areas of intracellular lipid collection in both macrophages and smooth muscle cells. Various concepts have been proposed to explain the progression of such precursor lesions to definite atherosclerosis [3, 4], most remarkable of which is the response-to-injury hypothesis postulating a cellular and molecular response to various atherogenic stimuli in the form of an inflammatory repair process [5]. This inflammation develops concurrently with the accumulation of minimally oxidized low density lipoproteins [6, 7], stimulates vascular smooth muscle cells (VSMCs), endothelial cells and macrophages [8], and as a result foam cells aggregate with an accumulation of oxidized LDL. In the further stages of artherosclerotic plaque development VSMCs migrate, proliferate, and synthesize extracellular matrix components on the luminal side of the vessel wall, forming the fibrous cap of the atherosclerotic lesion [9]. In this complex process of growth, progression, and finally rupture of an atherosclerotic plaque, a large number of matrix modulators, inflammatory mediators, growth factors, and vasoactive substances are involved. The complex interactions of these many factors are discussed in the special literature [6–10].
The fibrous cap of the atherosclerotic lesion covers the deep lipid core with a massive accumulation of extracellular lipids (atheromatous plaque), or fibroblasts and extracellular calcifications may contribute to a fibrocalcific lesion. Mediators from inflammatory cells at the thinnest portion of the cap surface of a vulnerable plaque – which is characterized by a larger lipid core and a thin fibrous cap – can lead to plaque disruption with formation of a thrombus or hematoma or even to total occlusion of the vessel. During the development of artherosclerosis the entire vessel can enlarge or constrict in size [11]. However, once the plaque covers >40% of the vessel wall, the artery no longer enlarges, and the lumen narrows as the plaque grows. In vulnerable plaques thrombosis forming on the disrupted lesion further narrows the vessel lumen and can lead to occlusion or be the origin of emboli. Less commonly, plaques have reduced collagen and elastin with a thin and weakened arterial wall, resulting in aneurysm formation which when ruptured may be the source of intracerebral hemorrhage (Figure 1.1).
Figure 1.1 The stages of development of an atherosclerotic plaque. (1) LDL moves into the subendothelium and (2) is oxidized by macrophages and smooth muscle cells (SMC). (3) Release of growth factors and cytokines (4) attracts additional monocytes. (5) Macrophages and (6) foam cell accumulation and additional (7) SMC proliferation result in (8) growth of the plaque. (9) Fibrous cap degradation and plaque rupture (collagenases, elastases). (10) Thrombus formation.
Injury hypothesis of progression to atherosclerosis: fatty streaks (focal areas of intracellular lipid collection) → inflammatory repair process with stimulation of vascular smooth muscle cells → atheromatous plaque.
Thromboembolism: Immediately after plaque rupture or erosion, subendothelial collagen, the lipid core, and procoagulants such as tissue factor and von Willebrand factor are exposed to circulating blood. Platelets rapidly adhere to the vessel wall through the platelet glycoproteins (GP) Ia/IIa and GP Ib/IX [12] with subsequent aggregation to this initial monolayer through linkage with fibrinogen and the exposed GP IIb/IIIa on activated platelets. As platelets are a source of nitrous oxide (NO), the resulting deficiency of bioactive NO, which is an effective vasodilator, contributes to the progression of thrombosis by augmenting platelet activation, enhancing VSMC proliferation and migration, and participating in neovascularization [13]. The activated platelets also release adenosine diphosphate (ADP) and thromboxane A2 with subsequent activation of the clotting cascade. The growing thrombus obstructs or even blocks the blood flow in the vessel. Atherosclerotic thrombi are also the source for embolisms, which are the primary pathophysiologic mechanism of ischemic strokes, especially from carotid artery disease or of cardiac origin.
Rupture or erosion of atheromatous plaques → adhesion of platelets → thrombus → obstruction of blood flow and source of emboli.
Small-vessel disease usually affects the arterioles and is associated with hypertension. It is caused by subendothelial accumulation of a pathological protein, the hyaline, formed from mucopolysaccharides and matrix proteins. It leads to narrowing of the lumen or even occlusion of these small vessels. Often it is associated with fibrosis, which affects not only arterioles, but also other small vessels and capillaries and venules. Lipohyalinosis also weakens the vessel wall predisposing for the formation of “miliary aneurysms.” Small-vessel disease results in two pathological conditions: status lacunaris (lacunar state) and status cribrosus (état criblé). Status lacunaris is characterized by small irregularly shaped infarcts due to occlusion of small vessels; it is the pathological substrate of lacunar strokes and vascular cognitive impairment and dementia. In status cribrosus small, round cavities develop around affected arteries due to disturbed supply of oxygen and metabolic substrate. These “criblures” together with miliary aneurysms are the sites of vessel rupture causing typical hypertonic intracerebral hemorrhages [14–17]. A second type of small-vessel disease is characterized by the progressive accumulation of congophilic, βA4 immuno-reactive, amyloid protein in the walls of small to medium-sized arteries and arterioles. Cerebral amyloid angiopathy is a pathological hallmark of Alzheimer’s disease and also occurs in rare genetically transmitted diseases, e.g. CADASIL and Fabry disease [18]. For a more detailed discussion of the etiology and pathophysiology of the various specific vascular disorders see [19–21].
Small-vessel disease: subendothelial accumulation of hyaline in arterioles.
Types of Cerebrovascular Disease
Numbers relating to the frequency of the different types of acute CVD are highly variable depending on the source of data. The most reliable numbers come from the in-hospital assessment of stroke in the Framingham study determining the frequency of completed stroke: 60% were caused by atherothrombotic brain infarction, 25.1% by cerebral embolism, 5.4% by subarachnoid hemorrhage, 8.3% by intracerebral hemorrhage, and 1.2% by undefined diseases. In addition, transient ischemic attacks accounted for 14.8% of the total cerebrovascular events [22]. Since the first Framingham reports, the rate of stroke death has declined by more than one-third, but the relative frequency distribution of completed stroke is essentially the same [23].
Ischemic strokes result from a critical reduction of regional cerebral blood flow lasting beyond a critical duration, and are caused by atherothrombotic changes of the arteries supplying the brain or by emboli from sources in the heart, the aorta, or the large arteries. The pathological substrate of ischemic stroke is ischemic infarction of brain tissue, the location, extension, and shape of which depend on the size of the occluded vessel, the mechanism of arterial obstruction, and the compensatory capacity of the vascular bed (Figure 1.2). Occlusion of arteries supplying defined brain territories by atherothrombosis or embolizations lead to territorial infarcts of variable size: they may be large – e.g. the whole territory supplied by the middle cerebral artery – or small, if branches of large arteries are occluded or if compensatory collateral perfusion – e.g. via the circle of Willis or leptomeningeal anastomoses – is efficient in reducing the area of critically reduced flow [15, 17]. In a smaller number of cases, infarcts can also develop at the borderzones between vascular territories, when several large arteries are stenotic and the perfusion in these “last meadows” cannot be constantly maintained above the critical threshold of morphological integrity [24]. Borderzone infarctions are a subtype of the low-flow or hemodynamically induced infarctions, which are the result of critically reduced cerebral perfusion pressure in far-downstream brain arteries. The more common low-flow infarctions affect subcortical structures within a vascular bed with preserved but marginal irrigation [25]. Lacunar infarcts reflect disease of the vessels penetrating the brain to supply the capsule, the basal ganglia, thalamus, and paramedian regions of the brainstem [26]. Most often they are caused by lipohyalinosis of deep arteries (small-vessel disease), less frequent causes are stenosis of the MCA stem and microembolization to penetrant arterial territories. Pathologically these lacunes are defined as small cystic trabeculated scars about 5 mm in diameter, but they are more often observed on magnetic resonance images where they are accepted as lacunes up to 1.5 cm diameter. The classic lacunar syndromes include pure motor, pure sensory, and sensorimotor syndromes, sometimes ataxic hemiparesis, clumsy hand, dysarthria, and hemichorea/hemiballism, but higher cerebral functions are not involved. A new classification of stroke subtypes is mainly oriented on the most likely cause of stroke: atherosclerosis, small-vessel disease, cardiac source, or other cause [27].
Figure 1.2 Topography of the most common types of cerebral infarcts: (1) anterior cerebral artery (left: total infarction, right: infarct of recurrent artery of Heubner); (2) anterior and middle cerebral arteries (left: with, right: without lenticulostriate arteries); (3) borderzone infarcts between anterior and middle cerebral arteries; (4) cystic infarcts (left: centrum ovale, right: caudate); (5) and (6) middle cerebral artery (5 left: total, right: cortical, 6 left: minimal, right: wedge-shaped); (7) end-artery and borderzone infarcts of the perforating branches of middle cerebral artery; (8) posterior cerebral artery (left: total, right: subtotal).
Territorial infarcts are caused by an occlusion of arteries supplying defined brain territories by atherothrombosis or embolizations.
Borderzone infarcts develop at the borderzone between vascular territories and are the result of a critically reduced cerebral perfusion pressure (low-flow infarctions).
Lacunar infarcts are mainly caused by small-vessel disease.
Hemorrhagic infarctions, i.e. “red infarcts” in contrast to the usual “pale infarcts,” are defined as ischemic infarcts in which varying amounts of blood cells are found within the necrotic tissue. The amount can range from a few petechial bleeds in the gray matter of cortex and basal ganglia to large hemorrhages involving the cortical and deep hemispheric regions. Hemorrhagic transformation frequently appears during the second and third phases of infarct evolution, when macrophages appear and new blood vessels are formed in tissue consisting of neuronal ghosts and proliferating astrocytes. However, the only significant difference between “pale” and “red infarcts” is the intensity and extension of the hemorrhagic component, since in at least two-thirds of all infarcts petechial hemorrhages are microscopically detectable. Macroscopically, red infarcts contain multifocal bleedings which are more or less confluent and predominate in cerebral cortex and basal ganglia which are richer in capillaries than the white matter [28]. If the hemorrhages become confluent intrainfarct hematomas might develop, and extensive edema may contribute to mass effects and lead to malignant infarction. The frequency of hemorrhagic infarctions (HIs) in anatomic studies ranged from 18% to 42% [29], with a high incidence (up to 85% of HIs) in cardioembolic stroke [30].
Mechanisms for hemorrhagic transformation are manifold and vary with regard to the intensity of bleeding. Petechial bleeding results from diapedesis rather than vascular rupture. In severe ischemic tissue vascular permeability is increased and endothelial tight junctions are ruptured. When blood circulation is spontaneously or therapeutically restored, blood can leak out of these damaged vessels. This can also happen with fragmentation and distal migration of an embolus (usually of cardiac origin) in the damaged vascular bed, explaining delayed clinical worsening in some cases. For the hemorrhagic transformation also the collateral circulation might have an impact: in some instances reperfusion via pial networks may develop with the diminution of peri-ischemic edema at borderzones of cortical infarcts. Risk of hemorrhage is significantly increased in large infarcts with mass effect supporting the importance of edema for tissue damage and the deleterious effect of late reperfusion. In some instances also the rupture of the vascular wall secondary to ischemia-induced endothelial necrosis might cause an intrainfarct hematoma. Vascular rupture can explain very early hemorrhagic infarcts and early intrainfarct hematoma (between 6 and 18 hours after stroke), whereas hemorrhagic transformation usually develops within 48 hours to 2 weeks.
Hemorrhagic infarctions (HI) are defined as ischemic infarcts in which varying amounts of blood cells are found within the necrotic tissue. They are caused by leakage from damaged vessels, due to increased vascular permeability in ischemic tissue or vascular rupture secondary to ischemia.
Intracerebral hemorrhage (ICH) occurs as a result of bleeding from an arterial source directly into the brain parenchyma and accounts for 5–15% of all strokes [31, 32]. Hypertension is the leading risk factor, but in addition advanced age, race, and also cigarette smoking, alcohol consumption, and high serum cholesterol levels have been identified. In a number of instances ICH occurs in the absence of hypertension usually in atypical locations. These causes include small vascular malformations, vasculitis, brain tumors, and sympathomimetic drugs (e.g. cocaine). ICH may also be caused by cerebral amyloid angiopathy and rarely is elicited by acute changes in blood pressure, e.g. due to exposure to cold. The occurrence of ICH is also influenced by the increasing use of anti-thrombotic and thrombolytic treatment of ischemic diseases of the brain, heart, and other organs [33, 34].
Spontaneous ICH occurs predominantly in the deep portions of the cerebral hemispheres (“typical ICH”) [35]. Its most common location is the putamen (35–50% of cases). The subcortical white matter is the second most frequent location (approximately 30%). Hemorrhages in the thalamus are found in 10–15%, in the pons in 5–12%, and in the cerebellum in 7% of cases [36]. Most ICHs originate from the rupture of small, deep arteries with diameters of 50–200 μm, which are affected by lipohyalinosis due to chronic hypertension. These small vessel changes lead to weakening of the vessel wall and miliary micro-aneurysm and consecutive small local bleedings, which might be followed by secondary ruptures of the enlarging hematoma in a cascade or avalanche fashion [37]. After active bleeding started it can continue for a number of hours with enlargement of hematoma that is frequently associated with clinical deterioration [38].
Putaminal hemorrhages originate from a lateral branch of the striate arteries at the posterior angle resulting in an ovoid mass pushing the insular cortex laterally and displacing or involving the internal capsule. From this initial putaminal-claustral location a large hematoma may extend to the internal capsule and lateral ventricle, into the corona radiata, and into the temporal white matter. Putaminal ICHs are considered the typical hypertensive hemorrhages.
Caudate hemorrhage, a less common form of bleeding from distal branches of lateral striate arteries, occurs in the head of the caudate nucleus. This bleeding early connects to the ventricle and usually involves the anterior limb of the internal capsule.
Thalamic hemorrhages can involve most of this nucleus and extend into the third ventricle medially and the posterior limb of the internal capsule laterally. The hematoma may press on or even extend into the midbrain. Larger hematomas often reach the corona radiata and the parietal white matter.
Lobar (white matter) hemorrhages originate at the cortico-subcortical junction between gray and white matter and spread along the fiber bundles most commonly in the parietal and occipital lobes. The hematomas are close to the cortical surface and usually not in direct contact with deep hemisphere structures or the ventricular system. As atypical ICHs they are not necessarily correlated with hypertension.
Cerebellar hemorrhages usually originate in the area of the dentate nucleus from rupture of distal branches of the superior cerebellar artery and extend into the hemispheric white matter and into the fourth ventricle. The pontine tegmentum is often compressed. A variant, the midline hematoma, originates from the cerebellar vermis, always communicates with the fourth ventricle, and frequently extends bilaterally into the pontine tegmentum.
Pontine hemorrhages from bleeding of small paramedian basilar perforating branches cause medially placed hematomas involving the basis of the pons. A unilateral variety results from rupture of distal, long circumferential branches of the basilar artery. These hematomas usually communicate with the fourth ventricle, and extend laterally and ventrally into the pons.
The frequency of recurrent ICHs in hypertensive patients is rather low (6%) [39]. Recurrence rate is higher with poor control of hypertension and also in hemorrhages due to other causes. In some instances multiple simultaneous ICHs may occur, but also in these cases the cause is another than hypertension.
In ICHs, the local accumulation of blood destroys the parenchyma, displaces nervous structures, and dissects the tissue. At the bleeding sites fibrin globes are formed around accumulated platelets. After hours or days extracellular edema develops at the periphery of the hematoma. After 4–10 days the red blood cells begin to lyse, granulocytes and thereafter microglial cells arrive, and foamy macrophages are formed, which ingest debris and hemosiderin. Finally, the astrocytes at the periphery of the hematoma proliferate and turn into gemistocytes with eosinophylic cytoplasma. When the hematoma is removed, the astrocytes are replaced by glial fibrils. After that period – extending to months – the residue of the hematoma is a flat cavity with a reddish lining resulting from hemosiderin-laden macrophages [36].
Intracerebral hemorrhage (ICH) occurs as a result of bleeding from an arterial source directly into the brain parenchyma, predominantly in the deep portions of the cerebral hemispheres (typical ICH). Hypertension is the leading risk factor, and the most common location is the putamen.
Cerebral venous thrombosis can develop from many causes and due to predisposing conditions. Cerebral venous thrombosis (CVT) is often multifactorial, when various risk factors and causes contribute to the development of this disorder [40]. The incidence of septic CVT has been reduced to less than 10% of cases, but septic cavernous sinus thrombosis is still a severe, however rare problem. Aseptic CVT occurs during puerperium and less frequently during pregnancy, but may also be related to use of oral contraceptives. Among the non-infectious causes of CVT congenital thrombophilia, particularly prothrombin and factor V Leiden gene mutations, as well as anti-thrombin, protein C, and protein S deficiencies must be considered. Other conditions with risk for CVT are malignancies, inflammatory diseases, and systemic lupus erythematosus. However, in 20–35% of CVT the etiology remains unknown. The fresh venous thrombus is rich in red blood cells and fibrin and poor in platelets. Later on, it is replaced by fibrous tissue, occasionally with recanalization. The most common location of CVT is the superior sagittal sinus and the tributary veins.
Whereas some thromboses, particularly of the lateral sinus, may have no pathological consequences for the brain tissue, occlusion of large cerebral veins usually leads to a venous infarct. These infarcts are located in the cortex and adjacent white matter and often are hemorrhagic. Thrombosis of the superior sagittal sinus may lead only to brain edema, but usually causes bilateral hemorrhagic infarcts in both hemispheres. These venous infarcts are different from arterial infarcts: cytotoxic edema is absent or mild, vasogenic edema is prominent, and hemorrhagic transformation or bleeding is usual. Despite this hemorrhagic component heparin is the treatment of choice.
Cerebral venous thrombosis can lead to a venous infarct. Venous infarcts are different from arterial infarcts: cytotoxic edema is absent or mild, vasogenic edema is prominent, and hemorrhagic transformation or bleeding is usual.
Cellular Pathology of Ischemic Injury
Acute interruption of cerebral blood flow causes a stereotyped sequel of cellular alterations which evolve over a protracted period of time and which depend on the topography, severity, and duration of ischemia [41]. Traditionally, these alterations have been studied by classical histological techniques, but recent developments in high resolution in vivo optical imaging such as multi-photon laser scanning microscopy (MPM), optical coherence tomography (OCT), or photoacoustic imaging (PAI) have opened the way to correlate morphological alterations with functional disturbances [42].
The most sensitive brain cells are neurons, followed – in this order – by oligodendrocytes, astrocytes, and vascular cells. The most vulnerable brain regions are hippocampal subfield CA1, neocortical layers 3, 5, and 6, the outer segment of striate nucleus, and the Purkinje and basket cell layers of cerebellar cortex. If blood flow decreases below the threshold of energy metabolism, the primary pathology is necrosis of all cell elements, resulting in ischemic brain infarct. If ischemia is not severe enough to cause primary energy failure, or if it is of so short duration that energy metabolism recovers after reperfusion, a delayed type of cell injury may evolve which exhibits the morphological characteristics of necrosis, apoptosis, necroptosis, or other forms of programmed cell death [43]. In the following, primary and delayed cell death will be described separately.
Primary Neuronal Cell Death
In the core of the territory of an occluded brain artery the earliest sign of cellular injury is neuronal swelling or shrinkage, the cytoplasm exhibiting microvacuolation (MV), which ultrastructurally has been associated with mitochondrial swelling [44]. These changes are potentially reversible if blood flow is restored before mitochondrial membranes begin to rupture. One to two hours after the onset of ischemia, neurons undergo irreversible necrotic alterations (red neuron or ischemic cell change [ICC]). In conventional hematoxilin-eosin stained brain sections such neurons are characterized by intensively stained eosinophilic cytoplasma, formation of triangular nuclear pyknosis, and direct contact with swollen astrocytes (Figure 1.3). Electron microscopically mitochondria exhibit flocculent densities, which represent denaturated mitochondrial proteins. Ischemic cell change must be distinguished from artifactual dark neurons, which stain with all (acid or basic) dyes and are not surrounded by swollen astrocytes [45].
Figure 1.3 Light-microscopical evolution of neuronal changes after experimental middle cerebral occlusion.
With ongoing ischemia, neurons gradually lose their stainability with hematoxilin, they become mildly eosinophilic, and, after 2–4 days, transform to ghost cells with hardly detectable pale outline. Interestingly, neurons with ischemic cell change are mainly located in the periphery and ghost cells in the center of the ischemic territory, which suggests that manifestation of ischemic cell change requires some residual or restored blood flow, whereas ghost cells may evolve in the absence of flow [41].
Primary ischemic cell death induced by focal ischemia is associated with reactive and secondary changes. The most prominent alteration during the initial 1–2 hours is perivascular and perineuronal astrocytic swelling, after 4–6 hours the blood–brain barrier breaks down resulting in the formation of vasogenic edema, after 1–2 days inflammatory cells accumulate throughout the ischemic infarct, and within 1.5–3 months cystic transformation of the necrotic tissue occurs together with the development of a peri-infarct astroglial scar (Figure 1.4).
Figure 1.4 Transformation of acute ischemic alterations into cystic infarct. Note pronounced inflammatory reaction prior to tissue cavitation.
Delayed Neuronal Death
The prototype of delayed cell death is the slowly progressing injury of pyramidal neurons in CA1 sector of hippocampus after a brief episode of global ischemia [46]. In focal ischemia delayed neuronal death may occur in the periphery of cortical infarcts or in regions which have been reperfused before ischemic energy failure becomes irreversible. Cell death is also observed in distant brain regions, notably in substantia nigra and thalamus.
The morphological appearance of neurons during the interval between ischemia and the manifestation of delayed cell death exhibits a continuum that ranges from necrosis to apoptosis with all possible combinations of cytoplasmic and nuclear morphology that are characteristic for the two types of cell death [47]. In its pure form, necrosis combines karyorhexis with massive swelling of endoplasmic reticulum and mitochondria, whereas in apoptosis mitochondria remain intact and nuclear fragmentation with condensation of nuclear chromatin gives way to the development of apoptotic bodies. In hemorrhagic stroke lysed blood may induce ferroptosis, a particular form of iron-dependent cell death, which is characterized by lethal accumulation of lipid reactive oxygen species (ROS) [48].
A widely used histochemical method for the visualization of apoptosis is terminal deoxyribonucleotidyl transferase (TdT)-mediated dUTP-biotin nick-end labeling (TUNEL assay), which detects DNA strand breaks. However, as this method may also stain necrotic neurons, a clear differentiation is not possible [49].
A consistent ultrastructural finding in neurons undergoing delayed cell death is disaggregation of ribosomes, which reflects the inhibition of protein synthesis at the initiation step of translation [50]. Light microscopically, this change is equivalent to tigrolysis, visible in Nissl-stained material that corresponds to the dissociation of ribosomes from the rough endoplasmic reticulum. Disturbances of protein synthesis and the associated endoplasmic reticulum stress are also responsible for cytosolic protein aggregation and the formation of stress granules [51]. In the hippocampus, stacks of accumulated endoplasmic reticulum may become visible, but in other areas this is not a prominent finding.
Pathology of Neurovascular Unit
The classical pathology of ischemic injury differentiates between the sensitivity of the various cell types of brain parenchyma with the neurons as the most vulnerable elements. The molecular analysis of injury evolution, however, suggests that ischemia initiates a coordinated multi-compartmental response of brain cells and vessels, also referred to as the neurovascular unit [52]. This unit includes microvessels (endothelial cells, basal lamina matrix, astrocytic endfeet, pericytes, and circulating blood elements), the cell body and main processes of astrocytes, the nearby neurons together with their axons, and supporting cells, notably microglia and oligodendrocytes. It provides the framework for the bi-directional communication between neuron and supplying microvessel. Under physiological condition, the most prominent function is the neurovascular coupling for maintaining adequate supply of brain nutrients and clearance of waste products. Pathophysiological disturbances of microcirculation provoke bi-directional responses, possibly mediated by alterations in the matrix of the vascular and non-vascular compartments of the ischemic territory. Pericytes positioned between endothelial cells, astrocytes, and neurons assume a central role in this process and are critically involved in mechanisms of both injury and repair of the central nervous system [53].
Severe ischemia induces primary cell death due to necrosis of all cell elements. Not so severe or short-term ischemia induces delayed cell death with necrosis, apoptosis, or a combination of both. The neurovascular unit provides the conceptual framework for the propagation of injury from microvessels to neurons.
Repair
Brain infarcts produced by focal ischemia are seemingly irresolvable in agreement with Cajal’s classical statement that in the adult brain “everything may die, nothing may be regenerated.” This dogma was reversed by the discovery of three permanently neurogenic regions, i.e. the subventricular zone (SVZ), the subgranular zone (SGZ), and the posterior perireticular (PPr) area, which provide lifelong supply of newly generated neurons to the hippocampus and olfactory bulb. After stroke, neurogenesis increases in these areas, and some of the newly formed cells migrate into the infarct penumbra, differentiate into oligodendrocytes and mature neurons, and survive for at least several weeks [54]. Neurogenesis may also occur through the neurovascular unit. After ischemia pericytes strongly migrate into the peri-infarct surrounding and contribute to tissue repair by controlling neurogenesis, angiogenesis, and blood–brain barrier function [55].
Ischemia-induced neurogenesis is enhanced by growth factors, nitric oxide, inflammation, non-coding RNA, and various hormones and neurotransmitters, notably estradiol and dopamine, but it is repressed by activation of NMDA subtype of glutamate receptors. The functional consequences of spontaneous or drug-enhanced neurogenesis are modest, but optimism is building up for targeted interventions. Similarly, considerable expectations are placed on local or systemic transplantation of exogenous neural progenitor cells and on cerebral endothelial and bone marrow cells, particularly in combination with growth factors and/or strategies that permit recruitment of transplanted cells to the site of injury [56]. However, cell therapy carries the risk of tumorigenesis, and as major breakthroughs have not yet been achieved, further research is necessary to explore the actual potentials of stroke regenerative medicine [57].
Several brain regions may provide lifelong supply of newly generated neurons.
Pathophysiology
The evolution of stroke is a highly intricate process which can be differentiated into two consecutive phases: a rather straightforward early “plumbing” problem that arises from the interruption of blood flow and brain energy supply, and a much more complex cascade of secondary events which depends on the interaction between metabolic and functional disturbances, on the one hand, and between hemodynamic and molecular alterations, on the other. As this complexity is differently depicted in different experimental stroke models, the technical particularities of these models must be understood before attempts are made to translate experimental insights to the clinical setting.
Animal Models of Stroke
According to the Framingham study, 65% of strokes that result from vascular occlusion present lesions in the territory of the middle cerebral artery, 2% in the anterior, and 9% in the posterior cerebral artery territories. The remaining lesions are located in brainstem, cerebellum, or in watershed or multiple regions. For each of these stroke types specific experimental models have been developed [58], but in accordance with the dominant clinical incidence middle cerebral artery occlusion models are preferentially used in experimental stroke research.
Transorbital middle cerebral artery occlusion: This model was introduced for the production of stroke in monkeys [59], and later modified for use in cats, dogs, rabbits, and even rats. The procedure is technically demanding and requires microsurgical skills. The advantage of this approach is the possibility to expose the middle cerebral artery at its origin from the internal carotid artery without retracting parts of the brain. Vascular occlusion can thus be performed without the risk of brain trauma. On the other hand, removal of the eyeball is invasive and may evoke functional disturbances, which should not be ignored. Surgery may also cause generalized vasospasm, which may interfere with the collateral circulation and, hence, induce variations in infarct size. The procedure therefore requires extensive training before reproducible results can be expected.
The occlusion of the middle cerebral artery at its origin interrupts blood flow to the total vascular territory, including the basal ganglia, which are supplied by the lenticulo-striate arteries. These MCA branches are end-arteries, which in contrast to the cortical branches do not form collaterals with the adjacent vascular territories. As a consequence, the basal ganglia are consistently part of the infarct core, whereas the cerebral cortex exhibits a gradient of blood flow, which decreases from the peripheral towards the central parts of the vascular territory. Depending on the steepness of this gradient, a cortical core region with the lowest flow values in the lower temporal cortex is surrounded by a variably sized penumbra, which may extend up to the parasagittal cortex.
Transcranial occlusion of the middle cerebral artery: Post- or retro-orbital transcranial approaches for middle cerebral artery occlusion are mainly used in rats and mice because in these species the main stem of the artery appears on the cortical surface rather close to its origin from the internal carotid artery [60]. Permanent occlusion is usually carried out by ligation or coagulation, and transient occlusion by clipping or lifting the vessel with a hook [61]. In contrast to transorbital middle cerebral artery occlusion, transcranial models do not produce ischemic injury in the basal ganglia because the lenticulo-striate branches originate proximal to the occlusion site. Infarcts, therefore, are mainly located in the temporo-parietal cortex with a gradient of declining flow values from the peripheral to the central parts of the vascular territory.
Filament occlusion of the middle cerebral artery: The presently most widely used procedure for middle cerebral artery occlusion in rats and mice is the intraluminal filament occlusion technique, first described by Koizumi [62]. A nylon suture with an acryl-thickened tip is inserted into the common carotid artery and orthogradely advanced, until the tip is located at the origin of the middle cerebral artery. Modifications of the original technique include different thread types for isolated or combined vascular occlusion, adjustments of the tip size to the weight of the animal, poly-L-lysine coating of the tip to prevent incomplete middle cerebral artery occlusion, or the use of guide-sheaths to allow remote manipulation of the thread for occlusion during polygraphic recordings or magnetic resonance imaging.
The placement of the suture at the origin of the middle cerebral artery obstructs blood supply to the total MCA-supplying territory, including the basal ganglia. It may also reduce blood flow in the anterior and posterior cerebral arteries, particularly when the common carotid artery is ligated to facilitate the insertion of the thread. As this minimizes collateral blood supply from these territories, infarcts are very large and produce massive ischemic brain edema with a high mortality when experiments last for more than a few hours. For this reason, threads are frequently withdrawn after 1–2 hours following insertion. The resulting reperfusion salvages the peripheral parts of the MCA territory, and infarcts become smaller [63]. However, as the pathophysiology of transient middle cerebral artery occlusion differs from that of the clinically more relevant permanent occlusion models, the mechanisms of infarct evolution or the pharmacological responsiveness of the resulting lesions do not properly replicate that of clinical stroke [64].
Transient filament occlusion is also an inappropriate model for the investigation of spontaneous or thrombolysis-induced reperfusion. Withdrawal of the intraluminal thread induces instantaneous reperfusion, whereas spontaneous or thrombolysis-induced recanalization results in slowly progressing recirculation. As post-ischemic recovery is greatly influenced by the dynamics of reperfusion, outcome and pharmacological responsiveness of experimental transient filament occlusion is also distinct from most clinical situations of reversible ischemia, where the onset of reperfusion is much less abrupt.
Clot embolism of middle cerebral artery: Middle cerebral artery embolism with autologous blood clots is a clinically highly relevant but inherently variable stroke model, which requires careful preparation and placement of standardized clots to induce reproducible brain infarcts [65]. The most reliable procedure for clot preparation is thrombin-induced clotting of autologous blood within calibrated tubings, which results in cylindrical clots that can be dissected in segments of equal length. Selection of either fibrin-rich (white) or fibrin-poor (red) segments influences the speed of spontaneous reperfusion and results in different outcome. Clots can also be produced in situ by microinjection of thrombin [66] or photochemically by ultraviolet illumination of the middle cerebral artery following injection of rose Bengal [67].
The main application of clot embolism is for the investigation of experimental thrombolysis. The drug most widely used is human recombinant tissue plasminogen activator [68], but the dose required in animals is much higher than in humans; this must be remembered when possible side-effects such as t-PA toxicity are investigated. The hemodynamic effect, in contrast, is similar despite the higher dose and adequately reproduces the slowly progressing recanalization observed under clinical conditions.
A recent development of clinical stroke treatment and possibly the central challenge for future animal research is interventional thrombectomy [69, 70]. The animal most widely used for this research is the swine because the vessels are large enough to insert appropriate stent retriever devices, but as in this species the carotid access to the middle cerebral artery is impeded by a rete mirabile, clot embolism and retrieval is carried out via the internal maxillary or lingual artery [71]. Angiographic studies of this model confirm that clot retrieval by aspiration or removable stent devices results in immediate recanalization, but a detailed pathophysiological analysis of post-ischemic reperfusion and of the metabolic-functional recovery is not yet available. It has been suggested that the pathophysiology of thrombectomy can be replicated in small animals by technically simpler mechanical occlusion models, such as transient filament occlusion [72], but here again proper validation must be awaited.
Various procedures for artery occlusion models, mostly middle cerebral artery occlusion models, were developed to study focal ischemia in animals.
Stroke Genetics
Stroke is frequently the primary manifestation of a hereditary disorder [73]. The heritability of all ischemic strokes amounts to 37.9%, with differences between the various stroke subtypes (large vessel stroke 40.3%, small vessel stroke 16.1%, and cardioembolic stroke 32.6%). Early molecular genetic investigations relied on linkage studies or the examination of candidate genes, but with the advent of genome-wide association studies (GWAS) large numbers of single nucleotide polymorphisms (SNPs) are being examined in stroke patients [74]. These studies reveal an increasing number of genes involved in monogenic stroke syndromes or affecting the risk of common (sporadic) stroke. Examples of monogenic stroke syndromes are Cadasil (NOTCH3), Carasil or Maeda syndrome (HTRA1), Fabry disease, and MELAS. Genetic variants that affect the risk of common stroke are clearly related to different stroke subtypes and include the PTX2 and ZFHX3 genes which correlate with cardioembolic stroke in association with atrial fibrillation, or the HDAC9 and MMP12 genes which contribute to atherosclerosis-associated large vessel stroke. So far, no variants have been associated with small vessel stroke, but there is evidence for genetic influences on intracerebral hemorrhage, involving the APP, COL4A1, and APOE genes [75].
An important newly evolving research field is pharmacogenetics which investigates the genetic influence on the individual responsiveness to stroke therapy. Examples are genetic differences in the metabolism of anti-coagulants, notably dabigatran and clopidogrel, or of the fibrinolytic efficacy of rtPA. Such information is of substantial importance not only for individual drug selection and dosing, but also for decision-making between alternative therapies, such as endovascular versus thrombolytic treatment [76].
Hemodynamics
In the intact brain, cerebral blood flow is tightly coupled to the metabolic requirements of tissue (metabolic regulation), but remains essentially stable when blood perfusion pressure changes (autoregulation).
An important requirement for metabolic regulation is the vasodilatory responsiveness of blood vessels to carbon dioxide (CO2 reactivity), which can be tested by the application of carbonic anhydrase inhibitors or CO2 ventilation. Under physiological conditions, blood flow doubles when arterial pCO2 rises by about 30 mmHg, and is reduced by one-third when pCO2 declines by 15 mmHg. The vascular response to CO2 depends mainly on the changes of extracellular pH, but it is also modulated by other factors such as prostanoids, nitric oxide, and neurogenic influences.
Most of the energy generated by brain metabolism is used to cover the functional activity of the brain. As both are coupled locally to blood flow, regional alterations of flow and metabolism can be used for anatomical mapping of functional brain activity (neurovascular coupling).
Autoregulation of cerebral blood flow is the remarkable capacity of the vascular system to adjust its resistance in such a way that blood flow is kept constant over a wide range of cerebral perfusion pressures (80–150 mmHg). The range of autoregulation is shifted to the right, i.e. to higher values, in patients with hypertension and to the left during hypercarbia.
The mechanism of autoregulation is complex [77]. The dominating factor is a pressure-sensitive direct myogenic response initiated by the activation of stretch-sensitive cation channels of vascular smooth muscle. In addition, a flow-sensitive indirect smooth muscle response is initiated by changes in the shear stress of endothelial cells, which result in activation of various signal transduction pathways. Other influences are mediated by metabolic and neurogenic factors, but these may be secondary effects of lesser significance.
Metabolic regulation: cerebral blood flow is coupled to metabolic requirements of tissue by a vascular response to changes in CO2. Autoregulation: cerebral blood flow is kept constant over a wide range of cerebral perfusion pressures.
Disturbances of Flow Regulation
Focal cerebral ischemia is associated with tissue acidosis, which leads to vasoparalysis and, in consequence, to a severe disturbance of the metabolic regulation of blood flow. In the center of the ischemic territory, CO2 reactivity is abolished or even reversed, i.e. blood flow may decrease with increasing arterial pCO2. This paradoxical “steal” effect has been attributed to the rerouting of blood to adjacent non-ischemic brain regions in which CO2 reactivity remains intact.
Stroke also impairs autoregulation, but the disturbance is more severe at decreasing than at increasing blood pressure. This is explained by the fact that in the ischemic tissue a decrease of local brain perfusion pressure cannot be compensated by further vasorelaxation, whereas an increase may shift the local perfusion pressure into the autoregulatory range and cause vasoconstriction. An alternative explanation is “false autoregulation” due to brain edema because the increase in local tissue pressure prevents the rise of tissue perfusion. Failure of cerebral autoregulation can be demonstrated in such instances by dehydrating the brain in order to reduce brain edema.
When blood flow is progressively disturbed by gradual atherosclerotic obstruction of a brain artery, the local increase of vascular resistance is compensated by peripheral autoregulatory relaxation, provided the distal branches of the artery are not already fully dilated. The residual vasodilatory capacity is called hemodynamic reserve and can be evaluated by measuring the CO2 reactivity: the lower the flow response, the lesser the hemodynamic reserve and the greater the risk of brain ischemia [78].
After transient vascular obstruction, peripheral vasorelaxation persists for some time, which explains the phenomenon of post-ischemic hyperemia or luxury perfusion. During luxury perfusion, oxygen supply exceeds oxygen requirements of the tissue, as reflected by the appearance of red venous blood. With the cessation of tissue acidosis, vascular tone returns, and blood flow declines to or below normal. At longer recirculation times autoregulation – but not CO2 reactivity – may recover, resulting in failure of metabolic regulation. This is one of the reasons why primary post-ischemic recovery may be followed by delayed post-ischemic hypoperfusion and secondary metabolic failure [79].
Disturbances of flow regulation through ischemia: tissue acidosis leads to vasorelaxation, CO2 reactivity is abolished or even reversed, and autoregulation is impaired.
Microcirculatory Disturbances
With the increasing understanding of the pathobiology of the neurovascular unit the importance of microcirculatory disturbances for the evolution of ischemic brain injury has been recognized [80]. Such disturbances develop at the capillary level within the first hour of focal ischemia and may persist even after full reversal of vascular occlusion (no-reflow or incomplete microcirculatory reperfusion). The dominating pathology is the narrowing of the capillary lumen, induced by constriction or death of pericytes [81] and swelling of pericapillary astrocytic endfeet [82]. The capillaries are filled with aggregated red blood cells, leukocytes, and fibrin/platelet deposits, the high viscosity of which adds to the increased vascular resistance of the reduced capillary lumen.
The mechanism of microcirculatory impairment is multifactorial. Pericytes constrict in response to the generation of reactive oxygen species (ROS), swelling of astrocytic endfeet is due to cytotoxic brain edema, and leukocyte adhesion to the vessel wall is part of the inflammatory response mediated by the generation of chemoattractants, cytokines, and chemokines. Finally, the activation of proteolytic enzymes contributes to the dismantlement of basal lamina and results in damage of the blood–brain barrier, an increase in interstitial tissue pressure, and the risk of hemorrhagic transformation.
The impairment of microcirculation is equivalent to a reduction of nutritional blood flow. During permanent vascular occlusion, it aggravates the effect of primary ischemia, particularly in the borderzone of the infarct, and after transient vascular occlusion it may prevent adequate reoxygenation despite recanalization of the supplying artery. It is still unresolved to what extent microcirculatory impairment contributes to or originates from ischemic injury, but there is general consent that microvascular protection is a requirement for successful stroke treatment [83].
Focal brain ischemia is aggravated by microcirculatory disturbances, which may persist despite recanalization.
Collateral Circulation and Steal Phenomena
The brain is protected against focal disturbances of blood flow by the collateral circulation, which provides a subsidiary network of vascular channels when principal conduits fail [84]. The most important intracranial collateral circuits are the circle of Willis at the base of the skull which provides low resistance connections between the origins of the anterior, middle and posterior cerebral arteries, and Heubner’s network of leptomeningeal anastomoses which connects the distal cortical branches of these arteries. The functional efficacy of the collaterals critically determines the severity of an ischemic lesion both during permanent and following transient vascular occlusion [85].
In contrast to this protective effect, collaterals may also divert blood from one brain region to another, depending on the magnitude and direction of the blood pressure gradients across the anastomotic connections (for review, see [86]). The associated change of regional blood flow is called steal if it results in a decrease in flow, or inverse steal if it results in an improvement in flow. Inverse steal has also been referred to as the Robin Hood syndrome in analogy to the legendary hero who took from the rich and gave to the poor.
Steals are not limited to a particular vascular territory and may affect both the extra- and intracerebral circulation. Examples of extracerebral steals are the subclavian, the occipital-vertebral, and the ophthalmic steal syndrome. Intracerebral steal occurs across collateral pathways of brain, notably the circle of Willis and Heubner’s network of pial anastomoses. The pathophysiological importance of steal has been disputed, but as it depends on the individual hemodynamic situation, it may explain unintended effects when flow is manipulated by alterations of arterial pCO2 or vasoactive drugs. Most authors, therefore, do not recommend such manipulations for the treatment of stroke.
“Steal”: decrease in focal blood flow when blood is diverted from one brain region to another by anastomotic channels; “inverse steal” if that results in an improvement in flow.
Concept of Ischemic Penumbra
Opitz and Schneider [87] were the first to draw attention to the fact that an impairment of brain energy production induced by a reduction of blood flow affects the energy-consuming processes in a sequential way: first, the functional activity of the brain is impaired; followed, at a more severe degree of hypoperfusion, by the suppression of the metabolic activity required to maintain its structural integrity. The concept of two different thresholds of blood flow for the preservation of functional and structural integrity was later refined by Symon et al. [88], who used a model of focal ischemia to produce a range of reduced flow levels. These studies revealed that EEG and evoked potentials are disturbed at substantially higher flow rates than the breakdown of the potassium gradient across the plasma membranes (“anoxic depolarization”). Since the breakdown of this gradient reflects the loss of cell viability, Symon et al. suggested that neurons located in the flow range between “electrical” and “membrane” failure are functionally silent, but structurally intact. In focal ischemia, this flow range corresponds to a crescent-shaped region intercalated between the necrotic tissue and the normal brain; it has been termed “penumbra” in analogy to the partly illuminated area around the complete shadow of the moon in full eclipse [89]. In metabolic terms it is the decline of blood flow below the level required to cover the functional but not the structural energy requirements of the brain.
Energy Requirements of Brain Tissue
A normal adult male’s brain containing about 130 billion neurons (21.5 billion in the neocortex) [90] comprises only 2% of total body mass, yet consumes at rest approximately 20% of the body’s total basal oxygen consumption and 16% of the cardiac blood output. The brain’s oxygen consumption is used almost entirely for the oxidative metabolism of glucose which in normal physiological conditions is the almost exclusive substrate for the brain’s energy metabolism [91] (Table 1.1). Glucose metabolized – and energy delivered – to neuronal cell bodies is mainly to support cellular vegetative and house-keeping functions, e.g. axonal transport, biosynthesis of nucleid acids, proteins, lipids, as well as other energy-consuming processes not related directly to the generation of action potentials. Therefore, the rate of glucose consumption of neuronal cell bodies is essentially unaffected by neuronal functional activation. Increases in glucose consumption (and regional blood flow) evoked by functional activation are confined to synapse-rich regions, i.e. the neuropil, which contains axonal terminals, dendritic processes, and also the astrocytic processes that envelope the synapses. The magnitudes of these increases are linearly related to the frequency of action potentials in the afferent pathways, and increases in the projection zones occur regardless of whether the pathway is excitatory or inhibitory. Energy requirements of functional activation are due mostly to stimulation of the Na+K+-ATPase activity to restore the ionic gradients across the cell membrane and the membrane potentials following spike activity, and are rather high compared to the basal energy demands of neuronal cell bodies [92].
Table 1.1 Cerebral blood flow (CBF), oxygen utilization (CMRO2), and metabolic rates of glucose (CMRGIc) in man (approximated values)
Cortex | White matter | Global | |
---|---|---|---|
CBF (ml/100 g/min) | 65 | 21 | 47 |
CMRO2 | 230 | 80 | 160 |
(μmol/100 g/min) | |||
CMRGlc | 40 | 20 | 32 |
(μmol/100 g/min) |
In excitatory glutamatergic neurons, which account for 80% of the neurons in the mammalian cortex, glucose utilization during activation is mediated by astrozytes which consume glucose by anaerobic glycolysis to provide lactate to the neurons where it is used for oxidative metabolism [93] (Figure 1.5). Overall, 87% of the total energy consumed is required for signaling, mainly action potential propagation and post-synaptic ion fluxes, and only 13% is expended in maintaining membrane resting potential [94].
Figure 1.5 Schematic representation of the mechanism for glutamate-induced glycolysis in astrocytes during physiological activation.
The mechanisms by which neurotransmitters other than glutamate influence blood flow and energy metabolism in the brain are still poorly understood [95].
A normal adult male’s brain comprises only 2% of total body mass, yet consumes at rest approximately 20% of the body’s total basal oxygen consumption. Glucose is the almost exclusive substrate for the brain’s energy metabolism; 87% of the total energy consumed is required for signaling, mainly action potential propagation and post-synaptic ion fluxes.
Viability Thresholds of Brain Ischemia
The different amounts of energy required for the generation of membrane potential and the propagation of electrical activity are associated with different thresholds of blood flow that must be maintained to deliver the necessary amounts of oxygen and glucose from the blood to the brain. Functional activity – reflected by the amplitudes of spontaneous and evoked electrical activity – begins to decline at flow values below 50% of control and is completely suppressed at about 30% of control. In awake monkeys these values correspond to the progression of neurological injury from mild paresis at 22 ml/100g/min to complete paralysis at 8 ml/100g/min. Morphological damage evolves as soon as cell membranes depolarize (“terminal” depolarization) and occurs at flow values below 15–20% of control. Biochemically, functional suppression is associated with the inhibition of protein synthesis at about 50% and the development of lactacidosis at 30–40% of control, whereas membrane depolarization and morphological injury correspond to the breakdown of energy metabolism and the loss of ATP at about 18% of control (Figure 1.6).
Figure 1.6 Diagrammatic representation of viability thresholds of focal brain ischemia.
A detailed picture of the dynamics of injury evolution can be obtained by the simultaneous recording of local blood flow and spontaneous unit activity of cortical neurons [96] (Figures 1.7A, B). According to these measurements, unit activity disappears at a mean value of 18 ml/100g/min, but the large variability of the functional thresholds of individual neurons (6–22 ml/100g/min) indicates differential vulnerability even within small cortical sectors. This explains the gradual development of neurological deficits, which may be related to differences in single cell activity with regular or irregular discharges at flow levels above the threshold of membrane failure.
Figure 1.7 A: Activity of a single neuron during graded ischemia before, during, and after reversible MCA occlusion. B: Recovery of neuronal function after a limited period of ischemia. C: Diagram of CBF thresholds required for the preservation of function and morphology of brain tissue. The activity of individual neurons is blocked when flow decreases below a certain threshold (dashed line) and returns when flow is raised again above this threshold. The fate of a single cell depends on the duration for which CBF is impaired below a certain level. The solid line separates structurally damaged from functionally impaired, but morphologically intact tissue, the “penumbra.” The dashed line distinguishes viable from functionally impaired tissue.
Whereas neuronal function is impaired immediately when flow drops below this threshold, the development of irreversible morphological damage is time dependent. Based on unit recordings from a large number of neurons during and after ischemia of varying density and duration, it was possible to construct a discriminant curve representing the lowest residual blood flow and the longest duration of ischemia still permitting neuronal recovery (Figure 1.7C). These results broaden the concept of the ischemic penumbra: the tissue fate – potential of recovery or irreversible damage – is determined not only by the level of residual flow, but also by the duration of the flow disturbance. Each level of decreased flow can, on average, be tolerated for a defined period of time; as a rule flow between 17 and 20 ml/100g/min can be tolerated for prolonged periods, whereas flow rates of 12 ml/100g/min lasting for 2–3 hours result in infarction. However, as low-flow perfusion is inhomogeneous, individual cells may become necrotic after shorter periods of time and at higher levels of mean residual flow.
The ischemic penumbra is the range of perfusion between the flow threshold for preservation of function and the flow threshold for morphological integrity. It is characterized by the potential for functional recovery without morphological damage.
Imaging of Penumbra
Based on the threshold concept of brain ischemia, the penumbra can be localized on quantitative flow images using empirically established flow thresholds. A more reliable approach is the imaging of threshold-dependent biochemical and/or functional disturbances and the demarcation of the mismatch between disturbances which occur only in the infarct core and others which also affect the penumbra [97].
Under experimental conditions the most accurate method for the precise localization of the infarct core is the loss of ATP on bioluminescent images of tissue ATP content (Figure 1.8). A biochemical marker of core plus penumbra is tissue acidosis or the inhibition of protein synthesis. The penumbra is the difference between the respective lesion areas. The reliability of this approach is supported by the precise co-localization of gene transcripts that are selectively expressed in the penumbra, such as the stress protein Hsp70 or the documentation of the gradual disappearance of the penumbra with increasing ischemia time [98].
Figure 1.8 Biochemical imaging of infarct core, penumbra, and benign oligemia after experimental middle cerebral artery occlusion. The core is identified by ATP depletion, the penumbra by the mismatch between the suppression of protein synthesis and ATP depletion (top) or by the mismatch between tissue acidosis and ATP (bottom), and benign oligemia by the reduction of blood flow in the absence of biochemical alterations.
Non-invasive imaging of the penumbra is possible using positron emission tomography (PET) or magnetic resonance imaging (MRI) [99]. Widely used PET parameters are the increase in oxygen extraction or the mismatch between reduced blood flow and the preservation of vitality markers, such as flumazenil binding to central benzodiazepine receptors [100]. An alternative PET approach is the use of hypoxia markers such as 18F nitromidazol (F-MISO) which is trapped in viable hypoxic but not in normoxic or necrotic tissue [101].
The best established MRI approach for penumbra imaging is the calculation of mismatch maps between the signal intensities of perfusion (PWI) and diffusion-weighted images (DWI), but its reliability has been questioned [102]. An alternative method is quantitative mapping of the apparent diffusion coefficient (ADC) of water, which reveals a robust correlation with the biochemically characterized penumbra for ADC values between 90% and 77% of control [103]. Recently, MR stroke imaging has been performed by combining PWI, DWI, and pH-weighted imaging (pHWI) where the mismatch between DWI and pHWI detects the penumbra, and that between PWI and pHWI the area of benign oligemia, i.e. a region in which flow reduction is not severe enough to cause metabolic disturbances [104]. Diffusion kurtosis imaging (DKI), an extension of diffusion imaging, demarcates the regions with stroke-induced structural changes that provide information about acute and chronic alterations in the perilesional cortex [105].
Finally, new developments in non-invasive molecular and optical imaging are of increasing interest for stroke research [106]. Using specific fluorescent probes and reporter gene technologies, these methods trace gene transcription and detect intracellular conjugates that reflect the metabolic status and/or bind to stroke markers. The number of molecules that can be identified by these methods rapidly expands and greatly facilitates the regional analysis of stroke injury.
Non-invasive imaging of the penumbra is possible using positron emission tomography (PET) or magnetic resonance imaging (MRI).
Temporal Evolution of Stroke
Focal brain ischemia produces brain infarction along two basically different pathophysiologies, depending on the severity and duration of the primary flow reduction, on the one hand, and the dynamics of post-ischemic recirculation, on the other. During permanent vascular occlusion, the central core of the ischemic infarct, i.e. the region in which blood flow declines below the threshold of cell viability, gradually expands into the penumbra (Figures 1.7 and 1.9). This expansion is not due to the progression of ischemia because the activation of collateral blood supply and spontaneous thrombolysis tend to improve blood flow over time.
Figure 1.9 Relationship between peri-infarct spreading depressions (above) and infarct growth (below) during permanent focal brain ischemia induced by occlusion of the middle cerebral artery in rat. The effect of spreading depressions on electrical brain activity (EEG) and blood flow (LDF) are monitored by DC recording of the cortical steady potential, and infarct growth by MR imaging of the apparent diffusion coefficient (ADC) of brain water.
Infarct growth can be differentiated into three phases. During the acute phase tissue injury is the direct consequence of the ischemia-induced energy failure and the resulting terminal depolarization of cell membranes. At flow values below the threshold of energy metabolism, this injury is established within a few minutes after the onset of ischemia. During the subsequent subacute phase, the infarct core expands into the peri-infarct penumbra until, after 3–6 hours, core and penumbra merge. The reasons for this expansion are peri-infarct spreading depressions and a multitude of cell biological disturbances, collectively referred to as molecular cell injury. Moreover, a late phase of injury evolves after several days to weeks after the onset of ischemia. During this phase secondary phenomena such as vasogenic edema, inflammation, and possibly programmed cell death may contribute to a further progression of injury.
The largest increment of infarct volume occurs during the subacute phase in which the infarct core expands into the penumbra [107]. Using multiparametric imaging techniques for the differentiation between core and penumbra, evidence could be provided that in small rodents submitted to permanent occlusion of the middle cerebral artery, the penumbra equals the volume of the infarct core at 1 hour ischemia, but after 3 hours more than 50% and between 6 and 8 hours almost all of the penumbra has disappeared and is now part of the irreversibly damaged infarct core [98]. In larger animals infarct core may be heterogeneous with multiple mini-cores surrounded by multiple mini-penumbras, but also these lesions expand and eventually progress to a homogeneous defect with a similar time course [108].
After transient vascular occlusion stroke the pathophysiology of lesion growth is basically different. If blood flow is promptly restored within the first hour of ischemia, brain energy metabolism recovers throughout the ischemic territory including the infarct core. However, after a free interval of several hours or even days, a delayed type of neuronal death evolves in an area that roughly corresponds to the infarct core at the onset of ischemia. This injury differs from primary ischemic injury by the absence of major flow disturbances and, therefore, is mediated by molecular rather than hemodynamic variables, as during permanent ischemia.
Finally, if transient vascular occlusion is gradually reversed as during spontaneous or thrombolytically induced recanalization, breakdown of energy metabolism in the infarct core does not recover because blood flow is too low or too inhomogeneous to resuscitate the brain within the revival time of the tissue. However, the improvement of flow stabilizes the penumbra and interrupts the growth of the infarct core as soon as reperfusion starts.
During permanent vascular occlusion brain infarcts grow in three phases:
acute phase, within a few minutes after the onset of ischemia; terminal depolarization of cell membranes;
subacute phase, within 3–6 hours; molecular cell injury, the infarct core expands into the peri-infarct penumbra;
late phase, several days to weeks; vasogenic edema, inflammation, and possibly programmed cell death.
After transient vascular occlusion quality of reperfusion determines outcome:
abrupt reperfusion restores core metabolism, but may cause delayed neuronal cell death;
gradual reperfusion does not restore core injury, but alleviates infarct growth.
Mechanisms of Injury Progression
Spreading Depression
A functional disturbance contributing to the growth of the infarct core into the penumbra zone is the generation of peri-infarct spreading depression-like depolarizations [109] (Figure 1.10). These depolarizations are initiated at the border of the infarct core and spread over the entire ipsilateral hemisphere. During spreading depression the metabolic rate of the tissue markedly increases in response to the greatly enhanced energy demands of the activated ion exchange pumps [110]. In the healthy brain the associated increase of glucose and oxygen demands are coupled to a parallel increase of blood flow, but in the peri-infarct penumbra this flow response is suppressed or even reversed [111]. As a result, a misrelationship arises between the increased metabolic workload and the low oxygen supply, leading to transient episodes of hypoxia and the stepwise increase in lactate during the passage of each depolarization.
Figure 1.10 Schematic representation of molecular injury pathways leading to necrotic or apoptotic brain injury after focal brain ischemia. Injury pathways can be blocked at numerous sites, providing multiple approaches for the amelioration of both necrotic and apoptotic cell death.
The pathogenic importance of peri-infarct depolarizations for the progression of ischemic injury is supported by the linear relationship between the number of depolarizations and infarct volume. Correlation analysis of this relationship suggests that during the initial 3 hours of vascular occlusion each depolarization increases the infarct volume by more than 20%. This is probably one of the reasons that glutamate antagonists and other interventions, which inhibit the generation of spreading depolarizations, reduce the volume of brain infarcts [112].
Peri-infarct spreading depressions are depolarizations initiated at the border of the infarct core and may contribute to progression of ischemic injury.
Molecular Mechanisms
In the borderzone of permanent focal ischemia or within the ischemic territory after transient vascular occlusion, cellular disturbances may evolve that cannot be explained by a lasting impairment of blood flow or energy metabolism. These disturbances are referred to as molecular injury, where the term “molecular” does not anticipate any particular injury pathway (for reviews, see [113, 114]). The molecular injury cascades (Figure 1.10) are interconnected in complex ways, which makes it difficult to predict their relative pathogenic importance in different ischemia models. In particular, molecular injury induced by transient focal ischemia is not equivalent to the alterations that occur in the core or the penumbra of permanent ischemia. Therefore, the relative contribution of the following injury mechanisms differs in different types of ischemia.
Acidotoxicity: During ischemia oxygen depletion, the associated activation of anaerobic glycolysis causes an accumulation of lactic acid which depending on the severity of ischemia, blood glucose levels, and the degree of ATP hydrolysis results in a decline of intracellular pH to levels between 6.5 and below 6.0. As the severity of acidosis correlates with the severity of ischemic injury, it has been postulated that acidosis is neurotoxic. Evidence has also been provided that ASICs (acid-sensing ion channels) are glutamate-independent vehicles of calcium flux, and that blockade of ASICs attenuates stroke injury. This suggests that acidosis may induce calcium toxicity, and that this effect is the actual mechanism of acidotoxicity [115].
Excitotoxicity: Shortly after the onset of ischemia, excitatory and inhibitory neurotransmitters are released, resulting in the activation of their specific receptors. Among these neurotransmitters, particular attention has been attributed to glutamate, which under certain experimental conditions may produce excitotoxic cell death [116]. The activation of ionotropic glutamate receptors results in the inflow of calcium from the extracellular into the intracellular compartment, leading to mitochondrial calcium overload and the activation of calcium-dependent catabolic enzymes. The activation of metabotropic glutamate receptors induces the IP3-dependent signal transduction pathway, leading among others to the stress response of endoplasmic reticulum, and by induction of immediate-early-genes (IEG) to adaptive genomic expressions. At very high concentration, glutamate results in primary neuronal necrosis. However, following pharmacological inhibition of ionotropic glutamate receptors, an apoptotic injury mechanism evolves that may prevail under certain pathophysiological conditions.
The importance of excitotoxicity for ischemic cell injury has been debated, but this does not invalidate the beneficial effect of glutamate antagonists for the treatment of focal ischemia. An explanation for this discrepancy is the above-described pathogenic role of peri-infarct depolarizations in infarct expansion. As glutamate antagonists inhibit the spread of these depolarizations, the resulting injury is also reduced.
Calcium toxicity: In the intact cell, highly efficient calcium transport systems assure the maintenance of a steep calcium concentration gradient of approximately 1:10 000 between the extra- and the intracellular compartment on the one hand, and between the cytosol and the endoplasmic reticulum (ER) on the other. During ischemia, anoxic depolarization in combination with the activation of ionotropic glutamate and acid-sensing ion channels causes a sharp rise of cytosolic calcium [117]. At the onset of ischemia this rise is further enhanced by activation of metabotropic glutamate receptors which mediate the release of calcium from endoplasmic reticulum (ER), and after recovery from ischemia by activation of transient receptor potential (TRP) channels which perpetuate intracellular calcium overload despite the restoration of ion gradients (Ca2+ paradox) [118]. The changes in intracellular calcium activity are highly pathogenic. Prolonged elevation of cytosolic calcium causes mitochondrial dysfunction and induces catabolic changes, notably by activation of Ca2+-dependent effector proteins and enzymes such as endonucleases, phospholipases, protein kinases, and proteases that damage DNA, lipids, and proteins. The release of calcium from the ER evokes an ER stress response, which mediates a great number of ER-dependent secondary disturbances, notably inhibition of protein synthesis. Calcium-dependent pathological events are therefore complex and contribute to a multitude of secondary molecular injury pathways.
Free radicals: In brain regions with low or intermittent blood perfusion, reactive oxygen species (ROS) are formed which produce peroxidative injury of plasma membranes and intracellular organelles [119]. The reaction with nitric oxide leads to the formation of peroxynitrite, which also causes violent biochemical reactions. Secondary consequences of free radical reactions are the release of biologically active free fatty acids such as arachidonic acid, the induction of endoplasmic reticulum stress and mitochondrial disturbances, the initiation of an inflammatory response, breakdown of the blood–brain barrier, and fragmentation of DNA. The latter may induce apoptosis and thus enhance molecular injury pathways related to mitochondrial dysfunction. The therapeutic benefit of free radicals-targeted therapies, however, is limited and has to await the discovery of novel, more efficient drugs [120].
Nitric oxide toxicity: Nitric oxide (NO) is a product of NO synthase (NOS) acting on argenin. There are at least three isoforms of NOS: eNOS is constitutively expressed in endothelial cells, nNOS in neurons, and the inducible isoform iNOS mainly in macrophages. Pathophysiologically, NO has two opposing effects [121]. In endothelial cells the generation of NO leads to vascular dilation, an improvement of blood flow, and the alleviation of hypoxic injury, whereas in neurons it contributes to glutamate excitotoxicity and – by formation of peroxynitrite – to free radical-induced injury. The net effect of NO thus depends on the individual pathophysiological situation and is difficult to predict.
Zinc toxicity: Zinc is an essential catalytic and structural element of numerous proteins and a secondary messenger, which is released from excitatory synapses during neuronal activation. Cytosolic zinc overload may promote mitochondrial dysfunction and generation of reactive oxygen species (ROS), activate signal transduction pathways such as MAP kinase, enhance calcium toxicity, and promote apoptosis [122]. However, at low concentration zinc may also exhibit neuroprotective properties, indicating that cells may possess a specific zinc set-point by which too little or too much zinc can promote ischemic injury [123].
ER stress and inhibition of protein synthesis: A robust molecular marker for the evolution of ischemic injury is inhibition of protein synthesis, which persists throughout the interval from the onset of ischemia until cell death ensues [50]. It is initiated by a disturbance of the calcium homeostasis of the endoplasmic reticulum (ER), which results in ER stress and various cell biological abnormalities such as un- or misfolding of proteins, expression of stress proteins, and a global inhibition of the protein synthesizing machinery. The latter is due to the activation of protein kinase R (PKR), which causes phosphorylation and inactivation of the alpha subunit of eukaryotic initiation factor eIF2. This again leads to selective inhibition of polypeptide chain initiation, disaggregation of ribosomes, and inhibition of protein synthesis at the level of translation.
To restore ER function un- or misfolded proteins must be refolded (by activation of the unfolded protein response, UPR) or degraded (by ER-associated degradation, ERAD). Cells in which UPR and ERAD fail to restore ER function die by apoptosis [124].
Obviously, persistent inhibition of protein synthesis is incompatible with cell survival, but as the duration of inhibition does not correlate with the delay of cell death, other factors must also be involved.
Mitochondrial disturbances: To mitigate metabolic or environmental stress, functional mitochondria are maintained by fission and fusion [125]. During ischemia, the concurrence of an increased cytosolic calcium activity with the generation of reactive oxygen species may lead to an increase in permeability of the inner mitochondrial membrane (mitochondrial permeability transition, MPT), which has been associated with the formation of a permeability transition pore (PTP). The PTP is a Ca2+-, ROS (reactive oxygen species), voltage-dependent, and cyclosporine A–sensitive high-conductance channel, located in the inner mitochondrial membrane. It is also a reversible fast Ca2+ release channel, facilitated by the mitochondrial matrix protein cyclophilin D [126]. The increase in permeability of the inner mitochondrial membrane has two pathophysiologically important consequences. The breakdown of the electrochemical gradient interferes with mitochondrial oxidative phosphorylation and, in consequence, with aerobic energy production. Furthermore, the equilibration of mitochondrial ion gradients causes swelling of the mitochondrial matrix, which eventually will cause disruption of the outer mitochondrial membrane and the release of pro-apoptotic mitochondrial proteins into the cytosol (see below). Ischemia-induced mitochondrial disturbances thus contribute to delayed cell death both by impairment of the energy state and the activation of apoptotic injury pathways [127].
A large number of biochemical substrates, molecules, and mechanisms are involved in the progression of ischemic damage.
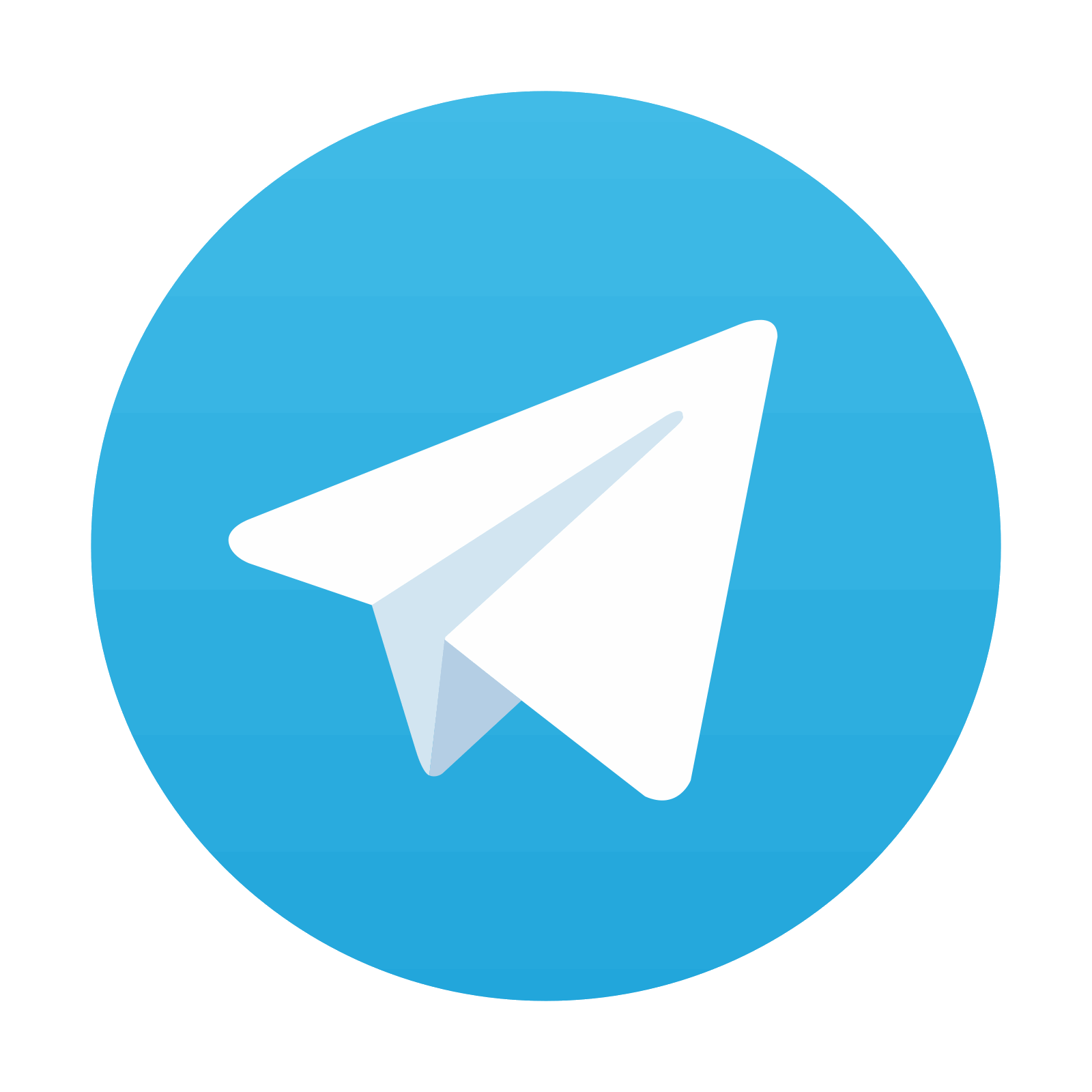
Stay updated, free articles. Join our Telegram channel
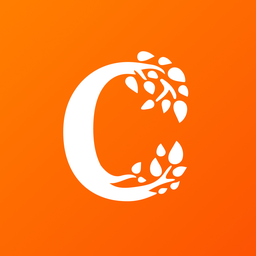
Full access? Get Clinical Tree
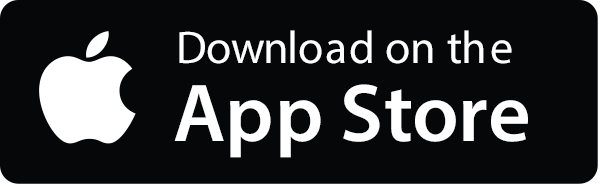
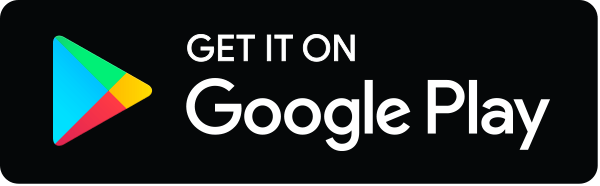
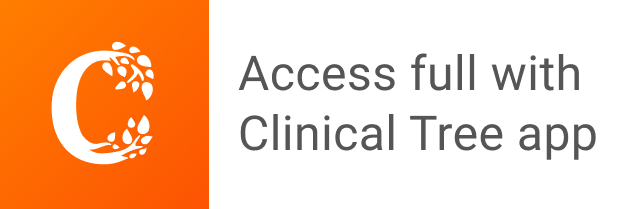